Miro proteins and their role in mitochondrial transfer in cancer and beyond
- 1Laboratory of Molecular Therapy, Institute of Biotechnology, Czech Academy of Sciences, Prague, Czechia
- 2Faculty of Science, Charles University, Prague, Czechia
- 3School of Pharmacy and Medical Science, Griffith University, Southport, QLD, Australia
Mitochondria are organelles essential for tumor cell proliferation and metastasis. Although their main cellular function, generation of energy in the form of ATP is dispensable for cancer cells, their capability to drive their adaptation to stress originating from tumor microenvironment makes them a plausible therapeutic target. Recent research has revealed that cancer cells with damaged oxidative phosphorylation import healthy (functional) mitochondria from surrounding stromal cells to drive pyrimidine synthesis and cell proliferation. Furthermore, it has been shown that energetically competent mitochondria are fundamental for tumor cell migration, invasion and metastasis. The spatial positioning and transport of mitochondria involves Miro proteins from a subfamily of small GTPases, localized in outer mitochondrial membrane. Miro proteins are involved in the structure of the MICOS complex, connecting outer and inner-mitochondrial membrane; in mitochondria-ER communication; Ca2+ metabolism; and in the recycling of damaged organelles via mitophagy. The most important role of Miro is regulation of mitochondrial movement and distribution within (and between) cells, acting as an adaptor linking organelles to cytoskeleton-associated motor proteins. In this review, we discuss the function of Miro proteins in various modes of intercellular mitochondrial transfer, emphasizing the structure and dynamics of tunneling nanotubes, the most common transfer modality. We summarize the evidence for and propose possible roles of Miro proteins in nanotube-mediated transfer as well as in cancer cell migration and metastasis, both processes being tightly connected to cytoskeleton-driven mitochondrial movement and positioning.
Introduction
Mitochondria are cellular powerhouses, generating most of the energy needed for metabolism of cells, as well as orchestrating a number of biochemical reactions from ATP production to amino acids generation or lipid biosynthesis. Except for these pathways, mitochondria regulate cell death, maintain calcium homeostasis and buffer reactive oxygen species (ROS). Mitochondria are highly dynamic organelles that constantly undergo cycles of fusion and fission, and, depending on the cellular type or metabolic status, they differ in size, shape or localization within the cell (Berridge et al., 2016; Furnish and Caino, 2020; Nahacka et al., 2021). They could be efficiently transported to the site of their need, utilizing the system of molecular motors together with tubulin and actin cytoskeleton (MacAskill and Kittler, 2010; Kittler, 2015; Eberhardt et al., 2020; Grossmann et al., 2020). Furthermore, mitochondria communicate with other organelles, often forming physical bridges with the endoplasmic reticulum (ER) or the nucleus (Modi et al., 2019; Desai et al., 2020).
For a long time it was thought that, mitochondria are not essential for cancer cells due to the dependence of tumor energy production on glycolysis (Warburg, 1956; Vander Heiden et al., 2009; Porporato et al., 2018; Vaupel et al., 2019; Pavlova et al., 2022). This misinterpretation has been corrected over the last decades, such that now we know that while mitochondrial ATP production is dispensable for tumorigenesis, mitochondria-linked pyrimidine biosynthesis is important to drive proliferation of cancer cells (Bajzikova et al., 2019; Boukalova et al., 2020). Our research as well as research of others has shown that cancer cells lacking mtDNA are able to form tumors only if they acquire respiration-competent mitochondria from neighboring cells of tumor stroma (Tan et al., 2015; Berridge et al., 2016; Dong et al., 2017; Bajzikova et al., 2019). Additionally, emerging evidence shows the importance of mitochondria in the process of tumor cell migration, invasion of distant tissues and formation of metastases (Jiang et al., 2012; Caino et al., 2016; Furnish and Caino, 2020; Ghosh et al., 2022).
In this short review, we will briefly discuss the role of Miro proteins in cancer and tumor microenvironment, especially in intercellular mitochondrial transfer from donor stromal cells to cancer cells focusing on tunneling nanotubes that are important for mitochondrial transfer between cells; the step that is necessary to initiate tumor cell proliferation and cancer progression during aberrant respiration. Additionally, we will also discuss the role of these proteins in spatial distribution of mitochondria and mitochondrial network, plus their role in the process of tumor cell invasion and metastasis. The comprehensive review of structure and functions of Miro1 and Miro2 is a focus of previous review articles by others and us (Nahacka et al., 2021). For more information, see Table 1.
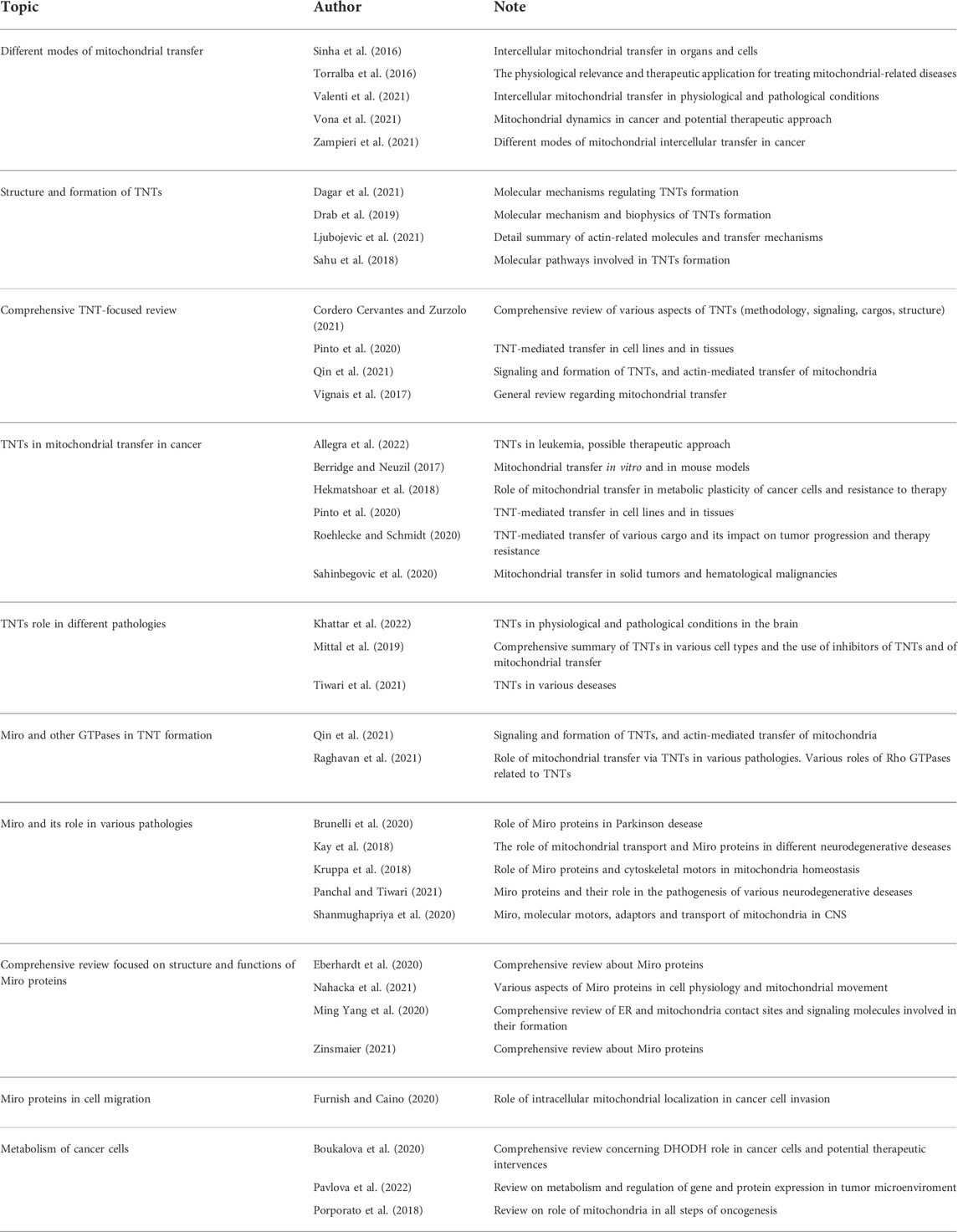
TABLE 1. Summary of review articles focusing on particular aspects of intercellular mitochondrial transfer, tunneling nanotubes and various functions of Miro proteins in physiological conditions and in different pathologies.
Miro proteins, their structure and function
Miro1 and Miro2 proteins (encoded by RHOT1 and RHOT2 genes, respectively) are main adaptors transporting mitochondria inside cells (Saotome et al., 2008; MacAskill et al., 2009a; Eberhardt et al., 2020). Miro proteins are evolutionary conserved throughout the eukaryotes with one homolog in yeasts (Gem1p) and lower metazoans (in D.melanogaster dMiro) and two homologs Miro1 and Miro2 in mammals (Frederick et al., 2004; Guo et al., 2005; Li X. et al., 2015; Beljan et al., 2020). These proteins classified as a specific subfamily of small GTPases comprise 620 amino acid residues and share 60% of sequence homology (Wennerberg and Der, 2004; Fransson et al., 2006; Reis et al., 2009). They are type II trans-membrane proteins embedded in the outer mitochondrial membrane consisting of the C-terminal trans-membrane domain and two canonical Ca2+ binding EF hand motifs flanked by two GTPase domains (the N-GTPase and the C-GTPase) (Fransson et al., 2006; MacAskill et al., 2009a; Reis et al., 2009; MacAskill and Kittler, 2010; Klosowiak et al., 2013). The C-terminal GTPase domain forms together with two EF-hand motifs, a rigid structure called MiroS (Klosowiak et al., 2013). While EF-hand domains feature calcium binding function, the two GTPase domains are both structurally and functionally distinct. It is thought that the more evolutionary conserved N-GTPase domain is of a greater importance for the function of the protein than the C-GTPase domain, supported by mutation studies showing a more severe impact on the phenotype in the case of the N-terminal domain mutation (Fransson et al., 2006; Reis et al., 2009; Smith et al., 2020). Except for nucleotide binding and GTP hydrolase activity of both domains, it was proposed that the C-GTPase domain possesses also nucleoside triphosphatase (NTPase) function (Peters et al., 2018; Smith et al., 2020). Miro exists in protein complexes, its interacting partners are mostly dimers or multimers (TRAK1/2, Kinesin-1, Mfn1/2), but the exact stoichiometry of Miro complexes needs to be elucidated. It was shown by crystallization studies that the N-GTPase domain has potential binding interfaces, which mediate homo-dimerization in the crystal (Smith et al., 2020).
Miro1 and Miro2 proteins are ubiquitously expressed with high level of Miro1 in the heart and in skeletal muscles and high level of Miro2 in the heart, liver, skeletal muscle, kidney and pancreas (UniProt Consortium, 2019). Despite their homology, they are able to compensate each other only to limited degree. During embryogenesis, both proteins are needed in different stages of development (Lopez-Domenech et al., 2016; Lopez-Domenech et al., 2018). Miro1 knock-out (Miro1 KO) is embryonically lethal while Miro2 knock-out (Miro2 KO) mice were found to develop normally until adulthood (Lopez-Domenech et al., 2016). Although Miro1 was able to compensate for the loss of Miro2, this did not happen in the opposite situation (Lopez-Domenech et al., 2016). Miro1 deletion led to disrupted mitochondrial trafficking and aberrant distribution of the organelles in dendritic neurons in vitro and in vivo, resulting in neurodegeneration.
Mitochondrial trafficking
It is known that Miro1 is a key regulator of mitochondrial trafficking not only in neurons but also in other cell types (Yamaoka et al., 2011; Kittler, 2015; Stephen et al., 2015; Lopez-Domenech et al., 2016; Lopez-Domenech et al., 2018; Kalinski et al., 2019; Eberhardt et al., 2020; Nahacka et al., 2021). Both proteins were found to bind protein complexes involved in tubulin (kinesin/dynein and TRAK1/2 complex) and actin (myosin XIX or Myo19) movement, but their participation in these processes is quite different (Brickley et al., 2005; Fransson et al., 2006; Glater et al., 2006; MacAskill et al., 2009a; Lopez-Domenech et al., 2018; Oeding et al., 2018; Bocanegra et al., 2020). As mentioned, Miro1 has a dominant function in mitochondrial localization and trafficking at long-range transport utilized by tubulin cytoskeleton (Lopez-Domenech et al., 2018). Bidirectional trafficking of mitochondria along microtubules is considered the dominant type of mitochondrial movement in metazoans (Schwarz, 2013). When observing anterograde and retrograde mitochondrial trafficking in hippocampal neurons or MEF cells in vitro, Miro2 KO did not have a significant effect while Miro1 KO decreased the extent of mitochondrial movement (Lopez-Domenech et al., 2018). Overexpression of Miro2 was not able to fully rescue the Miro1 KO phenotype (Lopez-Domenech et al., 2016; Lopez-Domenech et al., 2018). Even though ablation of the RHOT2 gene did not affect the localization of mitochondria in MEF cells, Miro2 protein was found to be partially involved in this process, since Miro1 and Miro2 double knock-out (DKO) accentuated phenotype (Lopez-Domenech et al., 2018). Still Miro2 cannot fully compensate for the loss of Miro1 and its role seems to be somewhat redundant in the presence of Miro1. Interestingly, both Miro proteins regulate actin-based movement with more dominant role of Miro2 in the short-range transport (Lopez-Domenech et al., 2018). Actin filaments were shown to be involved not only in short-range transport of mitochondria but also in dynamic processes such as fusion and fission or during mitosis in the proper segregation of mitochondria to daughter cells (Quintero et al., 2009; Korobova et al., 2014). The prevalent role of Miro2 in actin movement was reflected also in unequal segregation of mitochondria during mitosis (Lopez-Domenech et al., 2018). Miro proteins not only bind Myo19, the molecular motor moving mitochondria along actin filaments, but also stabilize it and protect it from degradation (Lopez-Domenech et al., 2016; Oeding et al., 2018). How Miro1 and Miro2 decide between different protein complexes when binding TRAK1/2 or Myo19 is still a question that needs to be answered. Interestingly, even in the absence of both Miro proteins a movement of mitochondria in a TRAK/kinesin-dependent manner could be detected (Lopez-Domenech et al., 2018). Most probably there are still unknown protein(s) anchoring mitochondria to tubulin at least for anterograde movement along tubulin fibers that is presumably also responsive to calcium regulation. Mitofusins Mfn1/2 could possibly play this role, since they were found to be bound in a complex with TRAK/Miro, and Mfn2 was shown to be necessary for axonal transport of mitochondria in D. melanogaster (Misko et al., 2010; Lee C. A. et al., 2018). Furthermore, in a recent study on C. elegans metaxins (MTX-1 and MTX-2), components of the protein import complex across the outer mitochondrial membrane (OMM), were identified as proteins capable to bind mitochondria to kinesin or dynein motors and bypass (but not fully recover) Miro failure (Zhao et al., 2021). Additionally, Myo19 was shown to be involved in Miro-independent interaction with mitochondria via its C-terminal membrane-association domain (MyMOMA) (Bocanegra et al., 2020).
Two main reservoirs of Ca2+ ions in cells are the endoplasmic reticulum and the mitochondria (Chang et al., 2011). Calcium is a general regulator of mitochondrial processes from trafficking to mitophagy, also affecting mitochondria-ER contact sites (MERCS) and processes such as apoptosis and mitochondrial bioenergetics (Wan et al., 1989; Saotome et al., 2008; MacAskill et al., 2009b; Stephen et al., 2015; Lee et al., 2016; Safiulina et al., 2019a; Safiulina et al., 2019b; Modi et al., 2019; Romero-Garcia and Prado-Garcia, 2019; Patergnani et al., 2020). Miro proteins have two EF-hand Ca2+-binding motifs. When the cytoplasmic concentration of Ca2+ increases, Miro binds calcium and changes its conformation that results in the release of the adaptor/motor complex from the cytoskeleton (Saotome et al., 2008; Klosowiak et al., 2013). Additionally, not only cytoplasmic but also mitochondrial calcium level probably impact on the frequency of mitochondria movement (Chang et al., 2011).
Miro as interacting partner of mitochondrial contact-site and cristae organizing system complex
Miro proteins are tethered in the OMM, and one can presume that their deletion will affect mitochondrial morphology and architecture. Both proteins were shown to be a part of the mitochondrial contact-site and cristae organizing system (MICOS) complex that connects OMM and the inner mitochondrial membrane (IMM) and that is important for the cristae architecture maintenance (Hoppins et al., 2011; Tsai et al., 2017; Modi et al., 2019; Yang M. et al., 2020; Stephan et al., 2020). Interestingly both Miro proteins equally cooperate in the regulation of mitochondrial morphology and, despite the changes in the shape of mitochondria and altered cristae organization; ablation of both genes does not affect the maximum respiratory capacity of the electron transport system in vitro (Lopez-Domenech et al., 2016; Modi et al., 2019). One of the reasons of this phenomenon could be that Miro DKO did not change the expression of components of the MICOS complex but rather altered its membrane distribution (Modi et al., 2019). More experiments are needed to see how Miro DKO affects the bioenergetics of cells and whether alteration of cristae architecture results in changes of the respiratory protein complexes composition.
In addition to movement of mitochondria along tubulin and actin cytoskeleton, Miro proteins were found to have a function in the process of mitophagy and regulation of MERCS (Kay et al., 2018; Eberhardt et al., 2020). Interestingly here they seem to be equally important and are able to mutually compensate each other suggesting that functional differences in mitochondrial trafficking would be more a question of different affinity to bind to molecular motors or to participate in different protein complexes involved in transport of mitochondria (Safiulina et al., 2019a; Safiulina et al., 2019b; Modi et al., 2019).
Mitophagy regulation
Mitophagy is a specific type of autophagy occurring in the case of energetic disbalance and defective mitochondria (Kittler, 2015; Pickles et al., 2018). One of the types of mitophagy was shown to be regulated by PINK1 kinase that activates Parkin, a ubiquitin ligase that ubiquitinates several downstream targets located in the OMM, including Miro proteins (Greene et al., 2012; Kane et al., 2014; Lazarou et al., 2015). These targets comprise, for example, a group of import receptors and ion channels such as TOM20, VDAC1 or VDAC3, and Mfn1 and Mfn2 proteins involved in the process of fusion and fission to block the fusion of defective mitochondria and Miro proteins (Geisler et al., 2010; Wang X. et al., 2011; Sun et al., 2012; Bingol et al., 2014; Birsa et al., 2014; Choubey et al., 2014). Parkin further facilitates K48 and K63 ubiquitination of substrates and recruits the autophagy machinery and complexes of lysosomal degradation (Olzmann et al., 2007; Moore et al., 2008; Richard et al., 2020; Park et al., 2021). Degradation of Miro by Parkin is a prerequisite for the arrest of mitochondrial movement, so that clearance of mitochondria can properly proceed (Wang X. et al., 2011; Birsa et al., 2014).
Miro proteins were found to be more than mere downstream targets for Parkin ubiquitination, themselves being active regulators of this process. Miro1 directly interacts with PINK1, whereby enhancing the catalytic activity of Parkin (Wang X. et al., 2011; Park et al., 2017). Moreover, both Miro proteins are required for proper Parkin translocation to OMM and for initiation of mitophagy (Safiulina et al., 2019a; Safiulina et al., 2019b). This process occurs independently of PINK1 activity or their own degradation and is regulated by calcium-binding ability of EF-hand domains of Miro proteins (Safiulina et al., 2019a; Safiulina et al., 2019b). Higher concentrations of calcium not only arrest mitochondrial movement by decreasing the affinity of Miro for its binding partners involved in mitochondrial transport but it also serves as a switch, recruiting (by Miro) cytosolic Parkin to mitochondria; priming mitochondria for the process of mitophagy (Yi et al., 2004; Saotome et al., 2008; Safiulina et al., 2019a; Safiulina et al., 2019b).
Dysregulation of mitophagy (and calcium signaling) is linked to many pathologies, starting with neurological diseases such as Parkinson disease (PD), heart failure, metabolic disorders, aging and cancer (Lahiri and Klionsky, 2017; Kay et al., 2018; Pickles et al., 2018; Cao et al., 2019; Kesharwani et al., 2019; Berenguer-Escuder et al., 2020; Brunelli et al., 2020; Eberhardt et al., 2020; Imai, 2020; Denisenko et al., 2021; Nahacka et al., 2021; Zhang et al., 2021; Babula and Krizanova, 2022; Diao and Gustafsson, 2022; Lee et al., 2022; Shan et al., 2022). Miro proteins are important regulators of the process of mitophagy, firstly prior to mitochondrial damage where they serve as calcium sensors, translocating Parkin to mitochondria, and then as Parkin downstream targets where ubiquitination of Miro proteins arrests mitochondrial trafficking needed for the organelle removal (Nahacka et al., 2021).
Involvement of Miro in mitochondria-ER contact sites
Connections between the endoplasmic reticulum and mitochondria (MERCS) have been described for single cell organisms as well as for metazoans (Vance, 1990; Frederick et al., 2004). MERCS are involved in many cellular functions. Both organelles serve as a reservoir of calcium; therefore, MERCS are involved in the regulation of calcium signaling (Rizzuto et al., 1998). Linked to this MERCS serve as a signaling hub for lipid metabolism, insulin signaling, mitochondrial fission, autophagy and apoptosis (Vance, 1990; Simmen et al., 2005; Friedman et al., 2011; Hamasaki et al., 2013; Tubbs et al., 2014; Sala-Vila et al., 2016; Rieusset, 2017). Gem1p, a homolog of Miro, was found to be a binding partner of ERMES (ER-mitochondria encounter structures) complex in yeasts (Frederick et al., 2004; Kornmann et al., 2011; Koshiba et al., 2011). A deletion study showed that the role of Gem1p is more in the regulation of ERMES than in the architecture of these structures (Kornmann et al., 2011). Interestingly calcium binding EF domains and N-GTPase domain were found necessary for the association of this protein with ERMES. Another example of Miro homolog with a function in mitochondria-ER contact sites (MERCS) was found in D. melanogaster (Lee et al., 2016; Lee K. S. et al., 2018). An important study showed that localization and interaction of dMiro with calcium transporters in MERCS and thus calcium homeostasis is dependent on phosphorylation of Miro by Polo kinase (Lee et al., 2016).
Interaction of Miro with calcium uniporters was shown also for humans (Niescier et al., 2013; Niescier et al., 2018). Calcium levels in Drosophila and the dMiro status were found to be important for proper brain development regardless of gain-of-function or loss-of-function mutation of dMiro (Lee et al., 2016; Lee K. S. et al., 2018). In both cases, mutations led to impaired calcium signaling that resulted either in activation of mitochondria-induced apoptosis or ATP depletion and cell growth inhibition, respectively. Polo kinase was shown to be important for brain development in not only lower organisms such as Drosophila, but its impaired function could also be found in humans in neurological diseases (e.g., PD) (Mbefo et al., 2010; Elfarrash et al., 2021; Weston et al., 2021).
Interestingly deregulated activity of this kinase was shown to be associated also with cancer (Naik et al., 2011; Craig et al., 2014). Other genes participating in the regulation of MERCS were shown to be related to PD in humans, such as PINK1, Parkin, leucine-rich repeat kinase 2 (LRRK2), α-synuclein and Miro. All of these genes are involved in regulation of mitochondrial quality control and mitophagy, processes connected by calcium signaling. Several studies showed a connection between RHOT1 gene mutations and disruption of Miro1 function in MERCS in PD patients (Berenguer-Escuder et al., 2019; Grossmann et al., 2019; Berenguer-Escuder et al., 2020; Grossmann et al., 2020). In all cases regardless of the type of mutation of RHOT1 gene, this resulted in the decrease of MERCS and eventually deregulated calcium signaling due to diminished connections between two organelles serving as reservoirs of calcium ions. Interestingly, gene-based association clustering methods revealed correlation between RHOT2 gene mutations and PD (Saeed, 2018). Moreover, equal contribution of Miro1 and Miro2 in regulation of MERCS was observed in MEFs isolated from knock-out mice (Modi et al., 2019). Re-expression of either Miro1 or Miro2 was able to rescue the WT phenotype, recover the extent of contacts between ER and mitochondria, and restore calcium signaling.
Miro proteins and different modes of mitochondrial transfer
The ability of cells to communicate via exchange of cellular components such as mitochondria is crucial for maintaining homeostasis in multicellular organisms. It has been shown that it also plays an important role in pathological states such as cancer, pulmonary disease, cardiomyopathy and brain damage (Islam et al., 2012; Tan et al., 2015; Hayakawa et al., 2016; Moschoi et al., 2016; Sinha et al., 2016; Torralba et al., 2016; Dong et al., 2017; Paliwal et al., 2018; Nicolas-Avila et al., 2020; Valenti et al., 2021; Vona et al., 2021; Zampieri et al., 2021). Mitochondrial transfer is triggered in response to various signals, most likely originating in the recipient cell; however, the molecular signals that initiate this “cross-talk” are still not fully clear.
There are several mechanisms of mitochondrial movement between cells, i.e., from the donor to the recipient cell. Tunneling nanotubes, actin-based cytoplasmic bridges, are generally perceived as the prevalent cellular structure that mediates intercellular mitochondrial transfer (Rustom et al., 2004; Gerdes and Carvalho, 2008; Vignais et al., 2017; Sahu et al., 2018; Drab et al., 2019; Cordero Cervantes and Zurzolo, 2021; Ljubojevic et al., 2021). Other proposed mechanisms include extracellular vesicles (EVs), which are secreted into the extracellular milieu by most cell types. EVs, ranging in size from 40 to 1,000 nm, can be divided into microvesicles, exosomes and apoptotic bodies, depending on their size, origin and molecular composition (Mittelbrunn and Sanchez-Madrid, 2012; Amari and Germain, 2021; Lazar and Goldfinger, 2021; Levoux et al., 2021). Interestingly, it was recently shown that EVs can be transported over longer distances as in inter-organ transport of mitochondria between energetically stressed adipocytes and cardiomyocytes (Crewe et al., 2021). Furthermore, the authors showed formation of mitochondria-derived vesicles (MDVs) that are packed into EVs and transport mitochondria to other cells (Crewe et al., 2021). It has been reported that Miro proteins, as part of outer mitochondrial membrane, are involved in the formation of MDVs (Konig et al., 2021). These particles are formed by budding of mitochondrial membranes and later fuse with either lysosomes or late endosomes (Soubannier et al., 2012). MDV forming was originally described for bacteria and serves as quality control of mitochondria (Soubannier et al., 2012; Pickles et al., 2018). Moreover, MDVs utilize communication between mitochondria and other organelles within the cells, especially with peroxisomes (Sugiura et al., 2017).
Other proposed mechanisms allowing for movement of mitochondria between cells include gap junctions and cell fusion. Gap junctions are clusters of intercellular channels that directly connect the cytoplasm of two adjacent cells allowing for direct diffusion of ions, small molecules and electrical pulses (Goodenough et al., 1996; Paliwal et al., 2018; Qin et al., 2021; Zampieri et al., 2021). Cell fusion is a process in which 2 cells fuse via their membranes, sharing organelles and cytosolic components, while nuclei remain intact (Aguilar et al., 2013; Valenti et al., 2021; Zampieri et al., 2021). Finally, mitochondrial extrusion is another proposed mechanism governing transfer of mitochondria between cells (Sinha et al., 2016; Torralba et al., 2016).
Notwithstanding the various modes of intercellular transfer of mitochondria, movement of the organelles between cells occurs in many cases via tunneling nanotubes, which is central for this review.
Tunneling nanotubes
Structure and formation of TNTs
TNTs were first described as several micrometers long actin-containing membrane connections formed between PC12 cells in vitro, mediating transfer of vesicles and organelle (Rustom et al., 2004). Since this initial observation, TNTs have been observed in a number of both cancer and non-cancerous cell types, both in vitro and in vivo (Islam et al., 2012; Jiang et al., 2016; Berridge and Neuzil, 2017; Hekmatshoar et al., 2018; Mittal et al., 2019; Pinto et al., 2020; Sahinbegovic et al., 2020; Tiwari et al., 2021; Allegra et al., 2022; Khattar et al., 2022). Although capable to move many different cargos (miRNA, lysosomes, liposomes, Golgi vesicles, calcium, etc.) across connections lasting for minutes to hours, most common cargo transferred via TNTs are mitochondria. Under physiological conditions, transfer of mitochondria from multipotent cells may serve as a mechanism of “rejuvenation” of the recipient cells, e.g., shifting them to more progenitor-like phenotype (Acquistapace et al., 2011). Inversely, transport of mitochondria from differentiated to multipotent cells can enhance their proliferation or trigger their differentiation into another cell type (Plotnikov et al., 2010; Vallabhaneni et al., 2012). Moreover, transfer of mitochondria was also demonstrated in differentiated cells between cardiomyocytes and cardiofibroblasts with TNTs being detected both in vitro and in the tissue (He et al., 2011).
In cancer, the formation of TNTs and intercellular exchange of mitochondria serves for maintaining the metabolic homeostasis of cancer cells and could be the reason of increased malignancy of cancer cells and cancer drug resistance (Moschoi et al., 2016; Rustom, 2016; Marlein et al., 2017; Hekmatshoar et al., 2018; Sahu et al., 2018; Marlein et al., 2019; Raghavan et al., 2021). One of the first pieces of evidences of TNT formation in malignant tissues comes from solid tumors isolated from patients with lung adenocarcinoma and pleural mesothelioma followed by similar observations in patient-derived ovarian and pancreatic cancer tissues (Lou et al., 2012b; Thayanithy et al., 2014; Desir et al., 2018). Later on, mitochondrial transfer via TNTs was observed in glioblastomas, multiple myelomas or in an in vitro model of breast cancer, laryngeal cancer, bladder cancer and others (Pasquier et al., 2013; Antanaviciute et al., 2014; Osswald et al., 2015; Lu et al., 2017; Marlein et al., 2019; Pinto et al., 2020; Salaud et al., 2020; D’Aloia et al., 2021; Pinto et al., 2021). Similarly, cancer cells have been shown to gain malignancy or enhanced resistance to drug treatment, hypoxia or apoptosis via TNTs (Pasquier et al., 2013; Ady et al., 2014; Wang and Gerdes, 2015; Desir et al., 2016; Rustom, 2016; Desir et al., 2018; Hekmatshoar et al., 2018; Sahu et al., 2018; Raghavan et al., 2021; Kato et al., 2022). Moreover, TNTs were identified in hematological malignancies, where inhibition of their formation improved the survival of diseased mice in vivo and enhanced chemosensitivity in vitro (Polak et al., 2015; Wang et al., 2018; Marlein et al., 2019). Interestingly, recent work illustrates that cancer cells transfer mitochondria via TNTs from lymphocytes, depleting immune cells on top of gaining metabolic advantage (Saha et al., 2022).
TNTs are classified as cell protrusions that 1) connect two (or more) cells; 2) do not touch the substrate; and 3) contain F-actin (Dupont et al., 2018; Roehlecke and Schmidt, 2020; Shanmughapriya et al., 2020). TNTs can connect cells of similar or different types, based on this they are referred to as homo- or hetero-TNTs (Dubois et al., 2020). Reported length of TNTs varies from tens to hundreds of micrometers and width from fifty to fifteen hundred nanometers (Ady et al., 2014; Austefjord et al., 2014; Dubois et al., 2020). There are two groups of TNTs, the first category includes thicker tubes (>700 nm) containing both tubulin and F-actin, whereas the second thinner tubes are composed of actin only (Sahu et al., 2018). This simple taxonomy was however recently challenged by the observation that thick TNTs formed by thin individual TNTs (or iTNTs) are bundled together by cadherin or spiraled up into thick TNTs (Sartori-Rupp et al., 2019; Franchi et al., 2020). Presence of intermediate filaments, the third main frequent cytoskeletal component, is much less understood as was reported in several studies, and most probably provides mechanical support (Ady et al., 2014; Resnik et al., 2018; Dubois et al., 2020; Latario et al., 2020).
There are several proposed mechanisms of TNT formation. They can start forming as filopodia or similar cellular protrusion from one of the cells, which will be connected with the other cell or they can form as residues of cell membranes after migration of cells away from each other (Sahu et al., 2018; Dubois et al., 2020; Roehlecke and Schmidt, 2020). As TNTs improve cell fitness under unfavorable conditions such as inflammatory environment, hypoxia or metabolic stress, their induction is connected to stress-related (p53, MAP kinase) and pro-survival (EGFR, Akt, PI3K or mTOR) signaling pathways promoting remodeling of the cell cytoskeleton and plasma membrane (Lou et al., 2012b; Ahmad et al., 2014; Desir et al., 2016; Sahu et al., 2018). Generally, there are three triggers resulting in TNT formation, often acting together: chemical or physical stressors (pH, hypoxia), inflammatory conditions (infection, toxins—LPS, or cytokines) and metabolic stress (starvation). Various inflammatory conditions have been demonstrated to induce TNTs such as LPS in cardiomyocytes or TNFα and IL-13 in epithelial cells. In both cases treatment induces the formation of more TNTs by the surrounding MSCs, which donate mitochondria (Ahmad et al., 2014; Yang Y. et al., 2020). In another study, RAGE receptors and subsequent MAP kinase signaling were shown to be involved in TNT formation in response to inflammatory conditions (Sun et al., 2012; Ranzinger et al., 2014). RAGE receptors are of exceptional interest as they are involved in both inflammation and oxidative stress responses, and they have been hypothesized to contribute to tissue homeostasis via TNT formation under various stress conditions (Rustom, 2016). Hypoxia, oxidative stress in the form of hydrogen peroxide and metabolic stress induced by serum depletion or mitochondrial damage induce or enhance TNT formation, illustrating that they present most likely a general mechanism utilized by distinct cell types in response to the various manifestations of mitochondrial malfunction (Wang Y. et al., 2011; Lou et al., 2012a; Lou et al., 2012b; Ahmad et al., 2014; Liu et al., 2014; Thayanithy et al., 2014; Desir et al., 2016; Jiang et al., 2016; Kretschmer et al., 2019; Yang Y. et al., 2020). Details of signaling molecules and pathways that bridge cellular stress and formation of TNTs are far from being elucidated, and our understanding is now rather contradictory. P53, a central hub of stress-related signaling pathways, was first described as essential for TNT formation, but later reported to be dispensable for this process (Wang Y. et al., 2011; Andresen et al., 2013; Zhang and Zhang, 2015). Other molecules reported to be involved in TNT formation include ROCK, PAK, MAP/ERK, PI3K, mTOR, and, thoroughly reviewed elsewhere (see Table 1).
Downstream from the above mentioned signaling pathways are proteins involved in TNT formation via interaction with the actin cytoskeleton or cell membrane. One such protein identified in several human and mouse cells is M-Sec (TNFAIP2), that regulates actin polymerization, filopodia formation and exocyst complex recruitment via interaction with small GTPases from the Rho (Cdc42) and Ral-like families (RalA) (Hase et al., 2009; Kimura et al., 2016; Lotfi et al., 2020; Barutta et al., 2021). Other proteins are the Arp2/3 complex branching actin, LST1 recruiting exocyst, RalA and actin cross-linking protein filamin or the unconventional motor protein Myo10 (Gousset et al., 2013; Schiller et al., 2013; Hanna et al., 2017; Tasca et al., 2017; Latario et al., 2020). Also, cell adhesion molecules and receptors (FAK, ICAM-1, or CD38) are frequently reported to be involved in TNT formation and maintenance or to correlate with mitochondrial transfer via TNTs (Sáenz-de-Santa-María et al., 2019; Wang et al., 2018; Marlein et al., 2019).
Mechanism of mitochondrial transfer via TNTs and the role of Miro proteins
By definition, all TNTs contain actin cytoskeleton and, in many cases, microtubules, both of these structures possibly acting as “tracks” for movement of mitochondria. Microtubule-associated motors dynein or kinesin mediate the movement of mitochondria towards minus or plus ends of microtubules over long distances, in contrast to short-range actin-mediated transport (MacAskill and Kittler, 2010). Miro proteins provide tethering of mitochondria to cytoskeletal motor proteins and serve as regulators of transport of the organelles, based on their calcium-dependent activity (Eberhardt et al., 2020; Nahacka et al., 2021). Essential role of Miro in intercellular transfer of mitochondria has been demonstrated by many studies reporting a correlation between Miro expression and mitochondria exchange with TNTs being the conduits for the transport (Ahmad et al., 2014; Babenko et al., 2018; English et al., 2020; Tseng et al., 2021; Wang et al., 2021). Except for one study, presence of Miro was found essential for the transfer/movement of mitochondria along TNTs rather than for their formation (Babenko et al., 2018; Raghavan et al., 2021). Although direct evidence connecting Miro to a particular alteration in TNT’s dynamic or structure is still missing, the process of TNTs formation via cytoskeleton and membrane remodeling is energetically demanding, with positioning of mitochondria (involving Miro) likely playing a role. It is still questionable, whether Miro proteins are more important for transport involving microtubules or actin structures as tracks for movement of mitochondria. Notwithstanding this unresolved question, given the notion that mitochondria move between cells over relatively long distances, it is more probable that Miro proteins are involved in tubulin-based transport. However, rather infrequent and contradictory reports regarding the importance of microtubules for TNT-mediated transfer between cells and reports of presence of mitochondria in TNTs lacking microtubules give rise to more questions that need to be answered (Luchetti et al., 2012; Wittig et al., 2012; Hanna et al., 2017; Sartori-Rupp et al., 2019).
Besides microtubule-associated motor proteins, Miro ensures direct interaction also with the actin motor protein myosin XIX that is hypothesized to be responsible for the movement of mitochondria via TNTs along actin filaments (Oeding et al., 2018; Qin et al., 2021). The same authors also mentioned the possibility of mitochondrial “docking” with myosin V or VI during their transition from the cytoplasm to TNTs. It would be interesting to find out whether this docking function of Miro proteins could be proved not only in the case of actin transport but also in the case of tubulin transport. It should be noted that mitochondria ought to “leave” TNTs on the side of the acceptor cells at their “+” termini and associate with tubulin fibers at their “+” termini. Mitochondria then move towards the “−” termini of cytoplasmic microtubules toward the perinuclear space, being propelled by dynein (see Figure 1). Here mitochondria can establish functional connection with recipient cellular organelles like the nucleus, ER or peroxisomes (Sugiura et al., 2017; Castro et al., 2018; Okumoto et al., 2018; Modi et al., 2019; Desai et al., 2020). Miro is involved not only in the “positioning” of mitochondria within recipient cells, but also in establishing functional connection of these organelles (Castro et al., 2018; Castro and Schrader, 2018; Okumoto et al., 2018; Modi et al., 2019; White et al., 2020; Guillen-Samander et al., 2021). Mitochondria from cancer cells could be possibly transported in opposite directions via TNTs (Caicedo et al., 2015; Lu et al., 2017). Exact regulation of mitochondrial movement directionality via Miro as well as the inner cytoskeletal organization of TNTs (e.g., orientation of microtubules) remains an intriguing topic for further investigation. Concerning damaged mitochondria, it is known that Miro plays an important role in the process of mitophagy, i.e., selective degradation of damaged mitochondria (Shlevkov et al., 2016; Lopez-Domenech et al., 2018; Safiulina et al., 2019a; Safiulina et al., 2019b). Moreover, Miro-assisted pulling of mitochondria along microtubules was recently described as a crucial step in the formation of mitochondrial-derived vesicles important for their quality control (Konig et al., 2021). Although much less common, intercellular transfer of damaged mitochondria has been demonstrated (Davis et al., 2014; Mahrouf-Yorgov et al., 2017; Wang et al., 2018; Crewe et al., 2021). In one case, Miro expression in recipient MSCs correlated with damage induced in donor cells by hydrogen peroxide (Mahrouf-Yorgov et al., 2017).
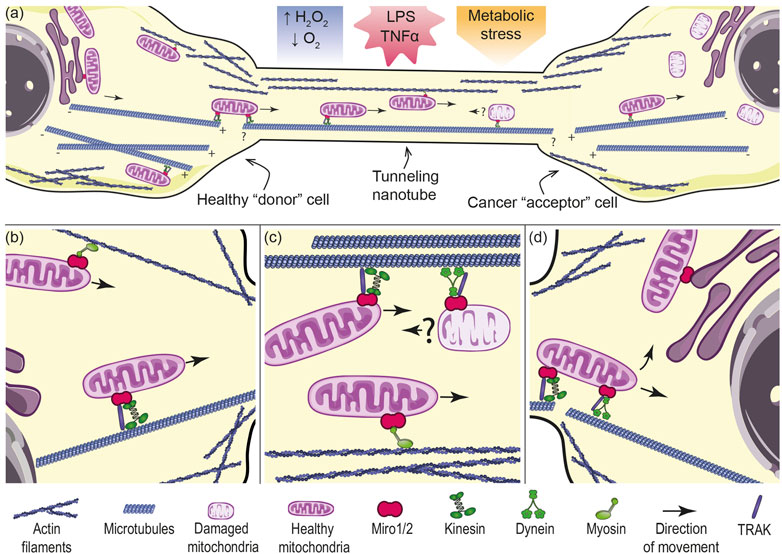
FIGURE 1. Mechanism of mitochondrial transport between two cells connected via TNTs. (A) Tunneling nanotube (TNT) formation between donor and acceptor cell could be pronounced by oxidative and metabolic stress or inflammatory conditions. Healthy mitochondria from the donor cell (left) move to a cancer cell (right) with damaged mitochondria. Mitochondria from cancer cells are possibly transported in opposite directions via TNTs. Mitochondrial transport in TNTs requires molecular motors that move the organelles along tubulin or actin filaments. It cannot be excluded that filaments are continuous from the donor cell into the TNT. (B) In the donor cell, mitochondria have to be transported towards the cell periphery into the base of TNTs. Peripheral localization of mitochondria is governed by kinesin or myosin motor proteins associated with microtubules or actin filaments respectively, tethered by Miro proteins. (C) Inside TNTs, healthy mitochondria are transported presumably along microtubules by kinesin motor with the assistance of TRAK adaptor and Miro, or along actin filaments by myosin with the assistance of Miro proteins. Damaged mitochondria could be possibly moved in the opposite direction by dynein with the assistance of Miro protein. (D) In the acceptor cell, imported mitochondria need to “switch the tracks” to be transported further within the cell. After import, mitochondria can establish functional connections with the nucleus (by means of nucleus-associated mitochondrial structures, NAMS), endoplasmic reticulum (ER-mitochondria encounter structures, ERMCS) and other organelles. The figure was created utilizing elements from Servier Medical Art (Creative Common Attribution 3.0 Generic License, https://smart.servier.com/).
Additionally Miro1 and partially Miro2 have been shown to be important for mitochondrial transport towards the cell periphery, especially in case of energy-demanding processes such as migration and invasion of cancer cells (Caino et al., 2016; Lopez-Domenech et al., 2018; Wang et al., 2021). Knock-out of these two proteins results in perinuclear localization of mitochondria, with more prominent phenotype in case of Miro1/Miro2 double knock-out (Kittler, 2015). It can be expected that successful displacement of mitochondria at the periphery of the donor cell is a necessary step for the process of mitochondrial transfer via TNTs; and, Miro proteins play an essential role in this process. As mentioned above, Miro proteins have also been shown to be part of the MICOS complex that connects outer mitochondrial membrane and inner mitochondrial membrane, whereby maintaining the integrity of cristae structure of mitochondria during their transfer (Modi et al., 2019).
The role of Miro proteins in cell migration and cancer metastasis
Recent findings show that localization of mitochondria within the cell is important for eventual cellular responses in the tumor niche (Senft and Ronai, 2016; Altieri, 2017; Alshaabi et al., 2020; Furnish and Caino, 2020). Constant reprogramming of cellular metabolism in order to meet the energetic demands of cancer cells requires a flexible system of mitochondrial trafficking. Distribution/positioning of functional mitochondria in cells can have an impact on energy-consuming processes such as proliferation, cell plasticity, migration and the subsequent metastatic capacity of cancer cells (Desai et al., 2013; Zhao et al., 2013; Caino et al., 2015; Rivadeneira et al., 2015; Furnish and Caino, 2020). During the process of migration and invasion of tumor cells, mitochondria are present in the cortical cytoskeleton and the whole mitochondrial network is positioned in the direction of the movement of the cells (Desai et al., 2013).
Morphological changes, changes of the cell shape and adhesion to extracellular matrix, together with the physical forces occurring during cell migration, are generated by F-actin cytoskeleton (Gardel et al., 2010). These processes are coordinated by a number of signaling events and biochemical reactions that have high demand for energy, which is provided by mitochondria. Similarly, the leading edge of migrating cells needs a supply of ATP that is produced by oxidative phosphorylation rather than by glycolysis, which is supported by re-distribution of mitochondria toward the leading edge regulated by AMP-activated protein kinase (Cunniff et al., 2016). The importance of ATP production at the leading edge of migrating cells was manifested in tumor cells with deficient oxidative phosphorylation (ρ0 cells lacking mtDNA) or in cells treated with inhibitors of mitochondrial respiratory complexes I-III and V (Caino et al., 2015).
Transport of mitochondria within cells occurs by means of their movement along actin and tubulin cytoskeleton with essential function of Miro proteins in this process. Several papers reported that inhibition of actin using specific inhibitors did not affect migration of the cells or focal adhesion formation, but inhibition of tubulin using nocodazol had a considerable effect on these processes, pointing to tubulin transport of mitochondria as playing an important role in positioning of mitochondria at the leading edge of migrating cells (Nguyen et al., 2018; Alshaabi et al., 2021). It was reported in several papers that knock-out of Miro1 results in perinuclear localization of mitochondria and in restricted mitochondrial network, but did not affect mitochondrial bioenergetics, most probably due to the compensatory mechanism of Miro2 as both proteins were shown to regulate mitochondrial morphology and cristae architecture (Nguyen et al., 2014; Cunniff et al., 2016; Schuler et al., 2017; Lopez-Domenech et al., 2018; Alshaabi et al., 2021).
An interesting work of Schuler et al. (2017) reported that intracellular position of mitochondria determines subcellular energy gradients with the highest ATP/ADP gradient in the perinuclear space that gradually declines towards the cell periphery and from the ventral to dorsal surface of the cell. These energy gradients correlate with the number and distribution of mitochondria. In Miro1−/− mouse embryonic fibroblasts (MEFs), this phenomenon was highly pronounced due to restricted mitochondrial localization. Knock-out of Miro1 led to increased level of ADP and reduced ATP/ADP ratio with the highest ratio in the area of highest mitochondrial density around the nucleus, rapidly declining towards the cell periphery. This change of intracellular energy distribution in Miro1−/− MEFs resulted in decreased leading edge protrusion and membrane ruffling, impaired focal adhesion formation and conclusively in reduced velocity of migrating cells (Schuler et al., 2017). Additionally, mitochondrial localization and density correlates not only with the ATP/ADP ratio but also with the level of hydrogen peroxide affecting local cellular responses to elevated mitochondrial ROS (Alshaabi et al., 2021). Consistent with this, it is known that the level of hydrogen peroxide is increased in the leading edge of migrating tumor cells (Cameron et al., 2015).
MEF cells lacking Miro1 have significantly reduced levels of peripheral hydrogen peroxide, which did not increase any further after inhibition of mitochondrial respiratory complex I with rotenone (Alshaabi et al., 2021). On the other hand, rotenone treatment induced higher level of nuclear hydrogen peroxide that was associated with elevated DNA damage response and correlated with perinuclear localization of the mitochondria in these cells (Alshaabi et al., 2021). These findings not only document that ATP and hydrogen peroxide (or ROS in general) are not simply diffused in the cell but form gradients copying the subcellular position of mitochondria, affecting the importance of the architecture of mitochondrial network and their distribution towards the “site of need” during highly energy demanding processes such as cellular migration. The importance of mitochondrial motility as an active part of the process of cell migration was demonstrated also in the model of atherosclerotic vascular disease in vascular smooth muscle cells (VSMCs) (Nguyen et al., 2018). Migration of VSMCs is regulated by mitochondrial Ca2+ calmodulin-dependent kinase II (mtCaMKII), the key regulator of the mitochondrial Ca2+ uniporter (MCU). Miro1 was found to be required for mtCaMKII-mediated mitochondrial translocation and Miro1 KO abolished migration of VSMCs cells (Nguyen et al., 2018).
Although the findings mentioned above were mostly acquired using non-cancerous cells (MEFs, VSMCs), several other papers implied the role of Miro proteins in the process of cell migration of cancer cells and their subsequent invasion and metastasis (Jiang et al., 2012; Desai et al., 2013; Li Q. et al., 2015; Caino et al., 2015; Caino et al., 2016; Mills et al., 2016; Qu et al., 2019; Wang et al., 2019; Furnish and Caino, 2020; Ghosh et al., 2022). Both proteins are expressed ubiquitously in various tissues and are not cancer-specific. RHOT1 gene expression can be found upregulated (pancreatic adenocarcinoma, cholangio carcinoma, esophageal carcinoma, glioblastoma or acute myeloid leukemia and thymoma) as well as downregulated (adrenocortical carcinoma, ovarian serous cystadenocarcinoma, skin cutaneous melanoma). On the other hand, RHOT2 is overall downregulated in cancer compared to normal tissue with exceptions being pancreatic adenocarcinoma, cholangio carcinoma and thymoma (as for Miro1) (Tang et al., 2017). However, relevant functional studies are missing. Several studies have shown a link between increased expression of Miro1 and Miro2 and cell migration or metastasis (Li Q. et al., 2015; Caino et al., 2016; Furnish et al., 2022). Expression of Miro1 was significantly higher in pancreatic ductal epithelial cells of pancreatic cancer from patients comparing to pre-cancerous tissues, and knock-down of Miro1 in SW1990 pancreatic adenocarcinoma cell line resulted in inhibition of cell migration (Li Q. et al., 2015). Interestingly, SMAD4 protein, a tumor-suppressor involved in TGFβ signaling, has been shown to be negatively regulated by Miro1 expression (Li Q. et al., 2015). Similarly, Miro2 expression was found to be significantly higher in several types of tumors compared to normal tissue and was upregulated in metastatic cancer cells when compared to primary tumors (Caino et al., 2016; Furnish et al., 2022). Downregulation of Miro1 expression was found to regulate negatively tumor cell invasion in syntaphilin knock-down cancer cells (Caino et al., 2016). Syntaphilin is a negative regulator of tumor cell migration and invasion, and the protein is connected with the tubulin transport of vesicles and was formerly thought to play a role exclusively in neurons; however, recent research revealed that it may be functional in other cell types (Caino et al., 2016; Caino et al., 2017; Seo et al., 2018; Hwang et al., 2019; Furnish and Caino, 2020; Chen et al., 2021). Although Miro2 did not have an effect on tumor cell invasion in the model of syntaphilin knock-down cells, it did effect, together with Miro1, mitochondrial trafficking and invasion of cancer cells in the model of stress induced tumor cell invasion, indicating a mechanism independent of the syntaphilin protein (Caino et al., 2016; Lopez-Domenech et al., 2018).
Discussion and conclusion
Miro proteins are important regulators of mitochondrial dynamics emanating their function beyond acting as mere adaptors that help movement of mitochondria along actin and tubulin fibers. Involvement in regulation of mitochondrial trafficking, regulation of mitophagy, an important tool of quality control of mitochondria, communication with other organelles such as peroxisomes, the endoplasmic reticulum or the nucleus, support of integrity of cristae structures as part of the MICOS complex, and regulation of calcium signaling that controls positioning of mitochondria within the cell, plus modulation of signaling pathways, makes the Miro proteins highly important players of regulation of mitochondrial metabolism and subsequent cellular responses (Yang M. et al., 2020; Eberhardt et al., 2020; Nahacka et al., 2021; Zinsmaier, 2021). In this review, we support the notion that mitochondria are indispensable for tumorigenesis and tumor progression (Porporato et al., 2018; Boukalova et al., 2020; Furnish and Caino, 2020). From in vitro and in vivo data we know that intercellular mitochondrial transfer could be found in tumors (Osswald et al., 2015; Tan et al., 2015; Moschoi et al., 2016; Dong et al., 2017; Bajzikova et al., 2019). Horizontal transfer of mitochondria between cells is a process that is still not well characterized, especially in cancer where detailed information is missing. The role of Miro in the process of intercellular mitochondrial transfer is supported in other disease models, where TNTs are involved (Kay et al., 2018; Kruppa et al., 2018; Panchal and Tiwari, 2021). Overexpression of Miro1 resulted in enhancement of horizontal transfer of mitochondria and improved cell recovery after ischemic or oxidative damage in different cell types (Ahmad et al., 2014; Babenko et al., 2018; Li et al., 2020; Tseng et al., 2021). Another paper showed that higher expression of Miro1 in iPSC-MSCs was responsible for superior efficacy in mitochondrial transfer compared to horizontal transfer of mitochondria observed in MSCs isolated from bone marrow in the model of cardiomyopathy (Zhang et al., 2016). This research points out to a rather general mechanism behind the regulation of mitochondrial “exchange” in different disease models, where Miro1 plays an important role in recovery of different types of cells after oxidative damage. Even though expression of both proteins Miro1 and Miro2 was found elevated in different types of patient tumors, crucial in vitro and in vivo data showing direct involvement of these proteins in the process of horizontal mitochondrial transfer in cancer are still missing. Available data we have are of an indirect character via involvement of Miro in the mitochondrial transport in TNTs (Qin et al., 2021; Raghavan et al., 2021).
As Miro2 is more redundant in the presence of Miro1 in terms of mitochondrial transport along tubulin and since it is not able to compensate for the loss of Miro1, it is highly probable that Miro2 would not be crucial for the process of horizontal transfer via TNTs and till this date Miro2 has not been documented to be involved in intercellular transfer of mitochondria. An example implying the possible role of Miro2 in horizontal transfer could be found in inter-mitochondrial communication in the heart, where Miro2 facilitates “mitochondrial nanotunneling” (Cao et al., 2019). Horizontal transfer of mitochondria is a complex process and even though Miro2 may not be a crucial player in the transfer of mitochondria per se, it could be important for the reconstruction of the mitochondrial network and re-establishing proper signalization (between mitochondria themselves or between ER and mitochondria) and finally respiration in the recipient cells. Since calcium signaling is fundamental for the mitochondrial function and both Miro1 and Miro2 have been found to equally contribute to calcium-regulated signaling (except for the mitochondrial trafficking), it is highly probable that the two proteins are equally involved in the process of re-establishing of mitochondrial function in the recipient cells.
As mentioned, TNTs are considered the most frequent means of intercellular mitochondrial transfer, but observation of their formation is limited to in vitro experiments. For this reason, further investigation of the formation and function of TNTs requires multimodal approaches. An example is the use of spatially defined co-cultures using microfluidic devices allowing quantitative assessment of TNTs formation as exemplified by the work of Yang et al. (2016). Ultrastructure analysis of TNTs, optimally using sophisticated microscopic methods such as FIB-SEM microscopy or CLEM, will be essential for the correct interpretation of structural details of TNTs as shown recently, providing surprising description of the TNT complex, involving smaller TNTs bundled together such that they appear as a single TNT (Sartori-Rupp et al., 2019).
We showed in our previous experimental work that mitochondrial transfer is necessary for tumor cell proliferation due to the role of mitochondria in de novo pyrimidine synthesis (Bajzikova et al., 2019). An interesting idea was proposed that cancer cells switch between the proliferative state to highly invasive and metastatic status by redistribution of mitochondria from the perinuclear space to the cortical cytoskeleton and the cell’s leading edge (Caino et al., 2017; Furnish and Caino, 2020). Recent data showing physical connections of the nucleus and mitochondria support this idea (Desai et al., 2020). Therefore, Miro proteins could play an important role in both mitochondrial transfer and cell invasion/metastasis. Redistribution of energetically active mitochondria to the cortical cytoskeleton was shown to be an important step for cancer cells to migrate and invade distant tissues. Even though the majority of data point to tubulin transport and Miro1 protein as crucial in the process of migration of cancer cells, as shown in the model of migration induced by PI3 kinase inhibitors, the involvement of actin transport and Miro2 remains questionable (Caino et al., 2016). More data are needed to elucidate the role of these two proteins in the process of migration and metastasis.
In this review, we discuss the notion that mitochondria and Miro proteins are essential in the two important phases of tumorigenesis. In cancer cells with aberrant oxidative phosphorylation, mitochondrial transfer is necessary to initiate tumor progression and, positioning of mitochondria within the cancer cell is crucial for further invasion and metastasis. This makes mitochondria an attractive target for translational medicine as already shown for chemotherapy of leukemia and radiotherapy of glioblastoma, where mitochondrial transfer to cancer cells from the stroma was documented to occur in the course of the treatment, possibly contributing to tumor resistance (Osswald et al., 2015; Moschoi et al., 2016).
Author contributions
ZN and JaN share first authorship. ZN and JiN wrote majority of the article. JaN wrote part Tunneling nanotubes, participated in discussion and put together Figure1 and Table 1. RZ participated in part Miro proteins and different modes of mitochondrial transfer. ZN and JiN contributed to the conception and design of the review. All authors corrected, reviewed and approved the final manuscript.
Funding
This study was supported by grants from the Czech Science Foundation (GA21-04607X and 20-05942S) to JiN, BIOCEV European Regional Development Fund CZ.1.05/1.100/02.0109 and the Institute of Biotechnology (RVO: 86652036). JaN was supported by a Grant Agency of Charles University (GAUK 320922) and MEYS CR (LM2018129 Czech-BioImaging).
Conflict of interest
The authors declare that the research was conducted in the absence of any commercial or financial relationships that could be construed as a potential conflict of interest.
Publisher’s note
All claims expressed in this article are solely those of the authors and do not necessarily represent those of their affiliated organizations, or those of the publisher, the editors, and the reviewers. Any product that may be evaluated in this article, or claim that may be made by its manufacturer, is not guaranteed or endorsed by the publisher.
References
Acquistapace, A., Bru, T., Lesault, P. F., Figeac, F., Coudert, A. E., le Coz, O., et al. (2011). Human mesenchymal stem cells reprogram adult cardiomyocytes toward a progenitor-like state through partial cell fusion and mitochondria transfer. Stem Cells 29 (5), 812–824. doi:10.1002/stem.632
Ady, J. W., Desir, S., Thayanithy, V., Vogel, R. I., Moreira, A. L., Downey, R. J., et al. (2014). Intercellular communication in malignant pleural mesothelioma: Properties of tunneling nanotubes. Front. Physiol. 5, 400. doi:10.3389/fphys.2014.00400
Aguilar, P. S., Baylies, M. K., Fleissner, A., Helming, L., Inoue, N., Podbilewicz, B., et al. (2013). Genetic basis of cell-cell fusion mechanisms. Trends Genet. 29 (7), 427–437. doi:10.1016/j.tig.2013.01.011
Ahmad, T., Mukherjee, S., Pattnaik, B., Kumar, M., Singh, S., Kumar, M., et al. (2014). Miro1 regulates intercellular mitochondrial transport & enhances mesenchymal stem cell rescue efficacy. EMBO J. 33 (9), 994–1010. doi:10.1002/embj.201386030
Allegra, A., Di Gioacchino, M., Cancemi, G., Casciaro, M., Petrarca, C., Musolino, C., et al. (2022). Specialized intercellular communications via tunnelling nanotubes in acute and chronic leukemia. Cancers (Basel) 14 (3), 659. doi:10.3390/cancers14030659
Alshaabi, H., Heininger, M., and Cunniff, B. (2020). Dynamic regulation of subcellular mitochondrial position for localized metabolite levels. J. Biochem. 167 (2), 109–117. doi:10.1093/jb/mvz058
Alshaabi, H., Shannon, N., Gravelle, R., Milczarek, S., Messier, T., Cunniff, B., et al. (2021). Miro1-mediated mitochondrial positioning supports subcellular redox status. Redox Biol. 38, 101818. doi:10.1016/j.redox.2020.101818
Altieri, D. C. (2017). Mitochondria on the move: Emerging paradigms of organelle trafficking in tumour plasticity and metastasis. Br. J. Cancer 117 (3), 301–305. doi:10.1038/bjc.2017.201
Amari, L., and Germain, M. (2021). Mitochondrial extracellular vesicles - origins and roles. Front. Mol. Neurosci. 14, 767219. doi:10.3389/fnmol.2021.767219
Andresen, V., Wang, X., Ghimire, S., Omsland, M., Gjertsen, B. T., Gerdes, H. H., et al. (2013). Tunneling nanotube (TNT) formation is independent of p53 expression. Cell. Death Differ. 20 (8), 1124. doi:10.1038/cdd.2013.61
Antanaviciute, I., Rysevaite, K., Liutkevicius, V., Marandykina, A., Rimkute, L., Sveikatiene, R., et al. (2014). Long-distance communication between laryngeal carcinoma cells. PLoS One 9 (6), e99196. doi:10.1371/journal.pone.0099196
Austefjord, M. W., Gerdes, H. H., and Wang, X. (2014). Tunneling nanotubes: Diversity in morphology and structure. Commun. Integr. Biol. 7 (1), e27934. doi:10.4161/cib.27934
Babenko, V. A., Silachev, D. N., Popkov, V. A., Zorova, L. D., Pevzner, I. B., Plotnikov, E. Y., et al. (2018). Miro1 enhances mitochondria transfer from multipotent mesenchymal stem cells (MMSC) to neural cells and improves the efficacy of cell recovery. Molecules 23 (3), 687. doi:10.3390/molecules23030687
Babula, P., and Krizanova, O. (2022). Involvement of calcium signaling in different types of cell death in cancer. Neoplasma 69 (2), 264–273. doi:10.4149/neo_2022_220127N121
Bajzikova, M., Kovarova, J., Coelho, A. R., Boukalova, S., Oh, S., Rohlenova, K., et al. (2019). Reactivation of dihydroorotate dehydrogenase-driven pyrimidine biosynthesis restores tumor growth of respiration-deficient cancer cells. Cell. Metab. 29 (2), 399–416. e310. doi:10.1016/j.cmet.2018.10.014
Barutta, F., Kimura, S., Hase, K., Bellini, S., Corbetta, B., Corbelli, A., et al. (2021). Protective role of the M-sec-tunneling nanotube system in podocytes. J. Am. Soc. Nephrol. 32 (5), 1114–1130. doi:10.1681/ASN.2020071076
Beljan, S., Herak Bosnar, M., and Cetkovic, H. (2020). Rho family of ras-like GTPases in early-branching animals. Cells 9 (10), E2279. doi:10.3390/cells9102279
Berenguer-Escuder, C., Grossmann, D., Antony, P., Arena, G., Wasner, K., Massart, F., et al. (2020). Impaired mitochondrial-endoplasmic reticulum interaction and mitophagy in Miro1-mutant neurons in Parkinson's disease. Hum. Mol. Genet. 29 (8), 1353–1364. doi:10.1093/hmg/ddaa066
Berenguer-Escuder, C., Grossmann, D., Massart, F., Antony, P., Burbulla, L. F., Glaab, E., et al. (2019). Variants in Miro1 cause alterations of ER-mitochondria contact sites in fibroblasts from Parkinson's disease patients. J. Clin. Med. 8 (12), E2226. doi:10.3390/jcm8122226
Berridge, M. V., McConnell, M. J., Grasso, C., Bajzikova, M., Kovarova, J., Neuzil, J., et al. (2016). Horizontal transfer of mitochondria between mammalian cells: Beyond co-culture approaches. Curr. Opin. Genet. Dev. 38, 75–82. doi:10.1016/j.gde.2016.04.003
Berridge, M. V., and Neuzil, J. (2017). The mobility of mitochondria: Intercellular trafficking in health and disease. Clin. Exp. Pharmacol. Physiol. 44 (Suppl. 1), 15–20. doi:10.1111/1440-1681.12764
Bingol, B., Tea, J. S., Phu, L., Reichelt, M., Bakalarski, C. E., Song, Q., et al. (2014). The mitochondrial deubiquitinase USP30 opposes parkin-mediated mitophagy. Nature 510 (7505), 370–375. doi:10.1038/nature13418
Birsa, N., Norkett, R., Wauer, T., Mevissen, T. E., Wu, H. C., Foltynie, T., et al. (2014). Lysine 27 ubiquitination of the mitochondrial transport protein Miro is dependent on serine 65 of the Parkin ubiquitin ligase. J. Biol. Chem. 289 (21), 14569–14582. doi:10.1074/jbc.M114.563031
Bocanegra, J. L., Fujita, B. M., Melton, N. R., Cowan, J. M., Schinski, E. L., Tamir, T. Y., et al. (2020). The MyMOMA domain of MYO19 encodes for distinct Miro-dependent and Miro-independent mechanisms of interaction with mitochondrial membranes. Cytoskelet. Hob. 77 (3-4), 149–166. doi:10.1002/cm.21560
Boukalova, S., Hubackova, S., Milosevic, M., Ezrova, Z., Neuzil, J., Rohlena, J., et al. (2020). Dihydroorotate dehydrogenase in oxidative phosphorylation and cancer. Biochim. Biophys. Acta. Mol. Basis Dis. 1866 (6), 165759. doi:10.1016/j.bbadis.2020.165759
Brickley, K., Smith, M. J., Beck, M., and Stephenson, F. A. (2005). GRIF-1 and OIP106, members of a novel gene family of coiled-coil domain proteins: Association in vivo and in vitro with kinesin. J. Biol. Chem. 280 (15), 14723–14732. doi:10.1074/jbc.M409095200
Brunelli, F., Valente, E. M., and Arena, G. (2020). Mechanisms of neurodegeneration in Parkinson's disease: Keep neurons in the PINK1. Mech. Ageing Dev. 189, 111277. doi:10.1016/j.mad.2020.111277
Caicedo, A., Fritz, V., Brondello, J. M., Ayala, M., Dennemont, I., Abdellaoui, N., et al. (2015). MitoCeption as a new tool to assess the effects of mesenchymal stem/stromal cell mitochondria on cancer cell metabolism and function. Sci. Rep. 5, 9073. doi:10.1038/srep09073
Caino, M. C., Ghosh, J. C., Chae, Y. C., Vaira, V., Rivadeneira, D. B., Faversani, A., et al. (2015). PI3K therapy reprograms mitochondrial trafficking to fuel tumor cell invasion. Proc. Natl. Acad. Sci. U. S. A. 112 (28), 8638–8643. doi:10.1073/pnas.1500722112
Caino, M. C., Seo, J. H., Aguinaldo, A., Wait, E., Bryant, K. G., Kossenkov, A. V., et al. (2016). A neuronal network of mitochondrial dynamics regulates metastasis. Nat. Commun. 7, 13730. doi:10.1038/ncomms13730
Caino, M. C., Seo, J. H., Wang, Y., Rivadeneira, D. B., Gabrilovich, D. I., Kim, E. T., et al. (2017). Syntaphilin controls a mitochondrial rheostat for proliferation-motility decisions in cancer. J. Clin. Invest. 127 (10), 3755–3769. doi:10.1172/JCI93172
Cameron, J. M., Gabrielsen, M., Chim, Y. H., Munro, J., McGhee, E. J., Sumpton, D., et al. (2015). Polarized cell motility induces hydrogen peroxide to inhibit cofilin via cysteine oxidation. Curr. Biol. 25 (11), 1520–1525. doi:10.1016/j.cub.2015.04.020
Cao, Y., Xu, C., Ye, J., He, Q., Zhang, X., Jia, S., et al. (2019). Miro2 regulates inter-mitochondrial communication in the heart and protects against TAC-induced cardiac dysfunction. Circ. Res. 125 (8), 728–743. doi:10.1161/CIRCRESAHA.119.315432
Castro, I. G., Richards, D. M., Metz, J., Costello, J. L., Passmore, J. B., Schrader, T. A., et al. (2018). A role for Mitochondrial Rho GTPase 1 (MIRO1) in motility and membrane dynamics of peroxisomes. Traffic 19 (3), 229–242. doi:10.1111/tra.12549
Castro, I. G., and Schrader, M. (2018). Miro1 - the missing link to peroxisome motility. Commun. Integr. Biol. 11 (4), e1526573. doi:10.1080/19420889.2018.1526573
Chang, K. T., Niescier, R. F., and Min, K. T. (2011). Mitochondrial matrix Ca2+ as an intrinsic signal regulating mitochondrial motility in axons. Proc. Natl. Acad. Sci. U. S. A. 108 (37), 15456–15461. doi:10.1073/pnas.1106862108
Chen, X., Xu, W., Zhuo, S., Chen, X., Chen, P., Guan, S., et al. (2021). Syntaphilin downregulation facilitates radioresistance via mediating mitochondria distribution in esophageal squamous cell carcinoma. Free Radic. Biol. Med. 165, 348–359. doi:10.1016/j.freeradbiomed.2021.01.056
Choubey, V., Cagalinec, M., Liiv, J., Safiulina, D., Hickey, M. A., Kuum, M., et al. (2014). BECN1 is involved in the initiation of mitophagy: It facilitates PARK2 translocation to mitochondria. Autophagy 10 (6), 1105–1119. doi:10.4161/auto.28615
Cordero Cervantes, D., and Zurzolo, C. (2021). Peering into tunneling nanotubes-The path forward. EMBO J. 40 (8), e105789. doi:10.15252/embj.2020105789
Craig, S. N., Wyatt, M. D., and McInnes, C. (2014). Current assessment of polo-like kinases as anti-tumor drug targets. Expert Opin. Drug Discov. 9 (7), 773–789. doi:10.1517/17460441.2014.918100
Crewe, C., Funcke, J. B., Li, S., Joffin, N., Gliniak, C. M., Ghaben, A. L., et al. (2021). Extracellular vesicle-based interorgan transport of mitochondria from energetically stressed adipocytes. Cell. Metab. 33 (9), 1853–1868. e11. doi:10.1016/j.cmet.2021.08.002
Cunniff, B., McKenzie, A. J., Heintz, N. H., and Howe, A. K. (2016). AMPK activity regulates trafficking of mitochondria to the leading edge during cell migration and matrix invasion. Mol. Biol. Cell. 27 (17), 2662–2674. doi:10.1091/mbc.E16-05-0286
D'Aloia, A., Arrigoni, E., Costa, B., Berruti, G., Martegani, E., Sacco, E., et al. (2021). RalGPS2 interacts with Akt and PDK1 promoting tunneling nanotubes formation in bladder cancer and kidney cells microenvironment. Cancers (Basel) 13 (24), 6330. doi:10.3390/cancers13246330
Dagar, S., Pathak, D., Oza, H. V., and Mylavarapu, S. V. S. (2021). Tunneling nanotubes and related structures: Molecular mechanisms of formation and function. Biochem. J. 478 (22), 3977–3998. doi:10.1042/BCJ20210077
Davis, C. H., Kim, K. Y., Bushong, E. A., Mills, E. A., Boassa, D., Shih, T., et al. (2014). Transcellular degradation of axonal mitochondria. Proc. Natl. Acad. Sci. U. S. A. 111 (26), 9633–9638. doi:10.1073/pnas.1404651111
Denisenko, T. V., Gogvadze, V., and Zhivotovsky, B. (2021). Mitophagy in carcinogenesis and cancer treatment. Discov. Oncol. 12 (1), 58. doi:10.1007/s12672-021-00454-1
Desai, R., East, D. A., Hardy, L., Faccenda, D., Rigon, M., Crosby, J., et al. (2020). Mitochondria form contact sites with the nucleus to couple prosurvival retrograde response. Sci. Adv. 6 (51), eabc9955. doi:10.1126/sciadv.abc9955
Desai, S. P., Bhatia, S. N., Toner, M., and Irimia, D. (2013). Mitochondrial localization and the persistent migration of epithelial cancer cells. Biophys. J. 104 (9), 2077–2088. doi:10.1016/j.bpj.2013.03.025
Desir, S., Dickson, E. L., Vogel, R. I., Thayanithy, V., Wong, P., Teoh, D., et al. (2016). Tunneling nanotube formation is stimulated by hypoxia in ovarian cancer cells. Oncotarget 7 (28), 43150–43161. doi:10.18632/oncotarget.9504
Desir, S., O'Hare, P., Vogel, R. I., Sperduto, W., Sarkari, A., Dickson, E. L., et al. (2018). Chemotherapy-induced tunneling nanotubes mediate intercellular drug efflux in pancreatic cancer. Sci. Rep. 8 (1), 9484. doi:10.1038/s41598-018-27649-x
Diao, R. Y., and Gustafsson, A. B. (2022). Mitochondrial quality surveillance: Mitophagy in cardiovascular health and disease. Am. J. Physiol. Cell. Physiol. 322 (2), C218–C230. doi:10.1152/ajpcell.00360.2021
Dong, L. F., Kovarova, J., Bajzikova, M., Bezawork-Geleta, A., Svec, D., Endaya, B., et al. (2017). Horizontal transfer of whole mitochondria restores tumorigenic potential in mitochondrial DNA-deficient cancer cells. Elife 6, e22187. doi:10.7554/eLife.22187
Drab, M., Stopar, D., Kralj-Iglic, V., and Iglic, A. (2019). Inception mechanisms of tunneling nanotubes. Cells 8 (6), E626. doi:10.3390/cells8060626
Dubois, F., Benard, M., Jean-Jacques, B., Schapman, D., Roberge, H., Lebon, A., et al. (2020). Investigating tunneling nanotubes in cancer cells: Guidelines for structural and functional studies through cell imaging. Biomed. Res. Int. 2020, 2701345. doi:10.1155/2020/2701345
Dupont, M., Souriant, S., Lugo-Villarino, G., Maridonneau-Parini, I., and Verollet, C. (2018). Tunneling nanotubes: Intimate communication between myeloid cells. Front. Immunol. 9, 43. doi:10.3389/fimmu.2018.00043
Eberhardt, E. L., Ludlam, A. V., Tan, Z., and Cianfrocco, M. A. (2020). Miro: A molecular switch at the center of mitochondrial regulation. Protein Sci. 29 (6), 1269–1284. doi:10.1002/pro.3839
Elfarrash, S., Jensen, N. M., Ferreira, N., Schmidt, S. I., Gregersen, E., Vestergaard, M. V., et al. (2021). Polo-like kinase 2 inhibition reduces serine-129 phosphorylation of physiological nuclear alpha-synuclein but not of the aggregated alpha-synuclein. PLoS One 16 (10), e0252635. doi:10.1371/journal.pone.0252635
English, K., Shepherd, A., Uzor, N. E., Trinh, R., Kavelaars, A., Heijnen, C. J., et al. (2020). Astrocytes rescue neuronal health after cisplatin treatment through mitochondrial transfer. Acta Neuropathol. Commun. 8 (1), 36. doi:10.1186/s40478-020-00897-7
Franchi, M., Piperigkou, Z., Riti, E., Masola, V., Onisto, M., Karamanos, N. K., et al. (2020). Long filopodia and tunneling nanotubes define new phenotypes of breast cancer cells in 3D cultures. Matrix Biol. Plus 6-7, 100026. doi:10.1016/j.mbplus.2020.100026
Fransson, S., Ruusala, A., and Aspenstrom, P. (2006). The atypical Rho GTPases Miro-1 and Miro-2 have essential roles in mitochondrial trafficking. Biochem. Biophys. Res. Commun. 344 (2), 500–510. doi:10.1016/j.bbrc.2006.03.163
Frederick, R. L., McCaffery, J. M., Cunningham, K. W., Okamoto, K., and Shaw, J. M. (2004). Yeast Miro GTPase, Gem1p, regulates mitochondrial morphology via a novel pathway. J. Cell. Biol. 167 (1), 87–98. doi:10.1083/jcb.200405100
Friedman, J. R., Lackner, L. L., West, M., DiBenedetto, J. R., Nunnari, J., Voeltz, G. K., et al. (2011). ER tubules mark sites of mitochondrial division. Science 334 (6054), 358–362. doi:10.1126/science.1207385
Furnish, M., Boulton, D. P., Genther, V., Grofova, D., Ellinwood, M. L., Romero, L., et al. (2022). MIRO2 regulates prostate cancer cell growth via GCN1-dependent stress signaling. Mol. Cancer Res. 20 (4), 607–621. doi:10.1158/1541-7786.MCR-21-0374
Furnish, M., and Caino, M. C. (2020). Altered mitochondrial trafficking as a novel mechanism of cancer metastasis. Cancer Rep. 3 (1), e1157. doi:10.1002/cnr2.1157
Gardel, M. L., Schneider, I. C., Aratyn-Schaus, Y., and Waterman, C. M. (2010). Mechanical integration of actin and adhesion dynamics in cell migration. Annu. Rev. Cell. Dev. Biol. 26, 315–333. doi:10.1146/annurev.cellbio.011209.122036
Geisler, S., Holmstrom, K. M., Skujat, D., Fiesel, F. C., Rothfuss, O. C., Kahle, P. J., et al. (2010). PINK1/Parkin-mediated mitophagy is dependent on VDAC1 and p62/SQSTM1. Nat. Cell. Biol. 12 (2), 119–131. doi:10.1038/ncb2012
Gerdes, H. H., and Carvalho, R. N. (2008). Intercellular transfer mediated by tunneling nanotubes. Curr. Opin. Cell. Biol. 20 (4), 470–475. doi:10.1016/j.ceb.2008.03.005
Ghosh, J. C., Perego, M., Agarwal, E., Bertolini, I., Wang, Y., Goldman, A. R., et al. (2022). Ghost mitochondria drive metastasis through adaptive GCN2/Akt therapeutic vulnerability. Proc. Natl. Acad. Sci. U. S. A. 119 (8), e2115624119. doi:10.1073/pnas.2115624119
Glater, E. E., Megeath, L. J., Stowers, R. S., and Schwarz, T. L. (2006). Axonal transport of mitochondria requires milton to recruit kinesin heavy chain and is light chain independent. J. Cell. Biol. 173 (4), 545–557. doi:10.1083/jcb.200601067
Goodenough, D. A., Goliger, J. A., and Paul, D. L. (1996). Connexins, connexons, and intercellular communication. Annu. Rev. Biochem. 65, 475–502. doi:10.1146/annurev.bi.65.070196.002355
Gousset, K., Marzo, L., Commere, P. H., and Zurzolo, C. (2013). Myo10 is a key regulator of TNT formation in neuronal cells. J. Cell. Sci. 126 (Pt 19), 4424–4435. doi:10.1242/jcs.129239
Greene, A. W., Grenier, K., Aguileta, M. A., Muise, S., Farazifard, R., Haque, M. E., et al. (2012). Mitochondrial processing peptidase regulates PINK1 processing, import and Parkin recruitment. EMBO Rep. 13 (4), 378–385. doi:10.1038/embor.2012.14
Grossmann, D., Berenguer-Escuder, C., Bellet, M. E., Scheibner, D., Bohler, J., Massart, F., et al. (2019). Mutations in RHOT1 disrupt endoplasmic reticulum-mitochondria contact sites interfering with calcium homeostasis and mitochondrial dynamics in Parkinson's disease. Antioxid. Redox Signal. 31 (16), 1213–1234. doi:10.1089/ars.2018.7718
Grossmann, D., Berenguer-Escuder, C., Chemla, A., Arena, G., and Kruger, R. (2020). The emerging role of RHOT1/Miro1 in the pathogenesis of Parkinson's Disease. Front. Neurol. 11, 587. doi:10.3389/fneur.2020.00587
Guillen-Samander, A., Leonzino, M., Hanna, M. G., Tang, N., Shen, H., De Camilli, P., et al. (2021). VPS13D bridges the ER to mitochondria and peroxisomes via Miro. J. Cell. Biol. 220 (5), e202010004. doi:10.1083/jcb.202010004
Guo, X., Macleod, G. T., Wellington, A., Hu, F., Panchumarthi, S., Schoenfield, M., et al. (2005). The GTPase dMiro is required for axonal transport of mitochondria to Drosophila synapses. Neuron 47 (3), 379–393. doi:10.1016/j.neuron.2005.06.027
Hamasaki, M., Furuta, N., Matsuda, A., Nezu, A., Yamamoto, A., Fujita, N., et al. (2013). Autophagosomes form at ER-mitochondria contact sites. Nature 495 (7441), 389–393. doi:10.1038/nature11910
Hanna, S. J., McCoy-Simandle, K., Miskolci, V., Guo, P., Cammer, M., Hodgson, L., et al. (2017). The role of rho-GTPases and actin polymerization during macrophage tunneling nanotube biogenesis. Sci. Rep. 7 (1), 8547. doi:10.1038/s41598-017-08950-7
Hase, K., Kimura, S., Takatsu, H., Ohmae, M., Kawano, S., Kitamura, H., et al. (2009). M-Sec promotes membrane nanotube formation by interacting with Ral and the exocyst complex. Nat. Cell. Biol. 11 (12), 1427–1432. doi:10.1038/ncb1990
Hayakawa, K., Esposito, E., Wang, X., Terasaki, Y., Liu, Y., Xing, C., et al. (2016). Transfer of mitochondria from astrocytes to neurons after stroke. Nature 535 (7613), 551–555. doi:10.1038/nature18928
He, K., Shi, X., Zhang, X., Dang, S., Ma, X., Liu, F., et al. (2011). Long-distance intercellular connectivity between cardiomyocytes and cardiofibroblasts mediated by membrane nanotubes. Cardiovasc. Res. 92 (1), 39–47. doi:10.1093/cvr/cvr189
Hekmatshoar, Y., Nakhle, J., Galloni, M., and Vignais, M. L. (2018). The role of metabolism and tunneling nanotube-mediated intercellular mitochondria exchange in cancer drug resistance. Biochem. J. 475 (14), 2305–2328. doi:10.1042/BCJ20170712
Hoppins, S., Collins, S. R., Cassidy-Stone, A., Hummel, E., Devay, R. M., Lackner, L. L., et al. (2011). A mitochondrial-focused genetic interaction map reveals a scaffold-like complex required for inner membrane organization in mitochondria. J. Cell. Biol. 195 (2), 323–340. doi:10.1083/jcb.201107053
Hwang, M. J., Bryant, K. G., Seo, J. H., Liu, Q., Humphrey, P. A., Melnick, M. A. C., et al. (2019). Syntaphilin is a novel biphasic biomarker of aggressive prostate cancer and a metastasis predictor. Am. J. Pathol. 189 (6), 1180–1189. doi:10.1016/j.ajpath.2019.02.009
Imai, Y. (2020). PINK1-Parkin signaling in Parkinson's disease: Lessons from Drosophila. Neurosci. Res. 159, 40–46. doi:10.1016/j.neures.2020.01.016
Islam, M. N., Das, S. R., Emin, M. T., Wei, M., Sun, L., Westphalen, K., et al. (2012). Mitochondrial transfer from bone-marrow-derived stromal cells to pulmonary alveoli protects against acute lung injury. Nat. Med. 18 (5), 759–765. doi:10.1038/nm.2736
Jiang, D., Gao, F., Zhang, Y., Wong, D. S., Li, Q., Tse, H. F., et al. (2016). Mitochondrial transfer of mesenchymal stem cells effectively protects corneal epithelial cells from mitochondrial damage. Cell. Death Dis. 7 (11), e2467. doi:10.1038/cddis.2016.358
Jiang, H., He, C., Geng, S., Sheng, H., Shen, X., Zhang, X., et al. (2012). RhoT1 and Smad4 are correlated with lymph node metastasis and overall survival in pancreatic cancer. PLoS One 7 (7), e42234. doi:10.1371/journal.pone.0042234
Kalinski, A. L., Kar, A. N., Craver, J., Tosolini, A. P., Sleigh, J. N., Lee, S. J., et al. (2019). Deacetylation of Miro1 by HDAC6 blocks mitochondrial transport and mediates axon growth inhibition. J. Cell. Biol. 218 (6), 1871–1890. doi:10.1083/jcb.201702187
Kane, L. A., Lazarou, M., Fogel, A. I., Li, Y., Yamano, K., Sarraf, S. A., et al. (2014). PINK1 phosphorylates ubiquitin to activate Parkin E3 ubiquitin ligase activity. J. Cell. Biol. 205 (2), 143–153. doi:10.1083/jcb.201402104
Kato, K., Nguyen, K. T., Decker, C. W., Silkwood, K. H., Eck, S. M., Hernandez, J. B., et al. (2022). Tunneling nanotube formation promotes survival against 5-fluorouracil in MCF-7 breast cancer cells. FEBS Open Bio 12 (1), 203–210. doi:10.1002/2211-5463.13324
Kay, L., Pienaar, I. S., Cooray, R., Black, G., and Soundararajan, M. (2018). Understanding Miro GTPases: Implications in the treatment of neurodegenerative disorders. Mol. Neurobiol. 55 (9), 7352–7365. doi:10.1007/s12035-018-0927-x
Kesharwani, R., Sarmah, D., Kaur, H., Mounika, L., Verma, G., Pabbala, V., et al. (2019). Interplay between mitophagy and inflammasomes in neurological disorders. ACS Chem. Neurosci. 10 (5), 2195–2208. doi:10.1021/acschemneuro.9b00117
Khattar, K. E., Safi, J., Rodriguez, A. M., and Vignais, M. L. (2022). Intercellular communication in the brain through tunneling nanotubes. Cancers (Basel) 14 (5), 1207. doi:10.3390/cancers14051207
Kimura, S., Yamashita, M., Yamakami-Kimura, M., Sato, Y., Yamagata, A., Kobashigawa, Y., et al. (2016). Distinct roles for the N- and C-terminal regions of M-sec in plasma membrane deformation during tunneling nanotube formation. Sci. Rep. 6, 33548. doi:10.1038/srep33548
Kittler, J. (2015). Regulation of mitochondrial trafficking, function and quality control by the mitochondrial GTPases Miro1 and Miro2. Springerplus 4 (Suppl. 1), L33. doi:10.1186/2193-1801-4-S1-L33
Klosowiak, J. L., Focia, P. J., Chakravarthy, S., Landahl, E. C., Freymann, D. M., Rice, S. E., et al. (2013). Structural coupling of the EF hand and C-terminal GTPase domains in the mitochondrial protein Miro. EMBO Rep. 14 (11), 968–974. doi:10.1038/embor.2013.151
Konig, T., Nolte, H., Aaltonen, M. J., Tatsuta, T., Krols, M., Stroh, T., et al. (2021). MIROs and DRP1 drive mitochondrial-derived vesicle biogenesis and promote quality control. Nat. Cell. Biol. 23 (12), 1271–1286. doi:10.1038/s41556-021-00798-4
Kornmann, B., Osman, C., and Walter, P. (2011). The conserved GTPase Gem1 regulates endoplasmic reticulum-mitochondria connections. Proc. Natl. Acad. Sci. U. S. A. 108 (34), 14151–14156. doi:10.1073/pnas.1111314108
Korobova, F., Gauvin, T. J., and Higgs, H. N. (2014). A role for myosin II in mammalian mitochondrial fission. Curr. Biol. 24 (4), 409–414. doi:10.1016/j.cub.2013.12.032
Koshiba, T., Holman, H. A., Kubara, K., Yasukawa, K., Kawabata, S., Okamoto, K., et al. (2011). Structure-function analysis of the yeast mitochondrial Rho GTPase, Gem1p: Implications for mitochondrial inheritance. J. Biol. Chem. 286 (1), 354–362. doi:10.1074/jbc.M110.180034
Kretschmer, A., Zhang, F., Somasekharan, S. P., Tse, C., Leachman, L., Gleave, A., et al. (2019). Stress-induced tunneling nanotubes support treatment adaptation in prostate cancer. Sci. Rep. 9 (1), 7826. doi:10.1038/s41598-019-44346-5
Kruppa, A. J., Kishi-Itakura, C., Masters, T. A., Rorbach, J. E., Grice, G. L., Kendrick-Jones, J., et al. (2018). Myosin VI-dependent actin cages encapsulate parkin-positive damaged mitochondria. Dev. Cell. 44 (4), 484–499. doi:10.1016/j.devcel.2018.01.007
Lahiri, V., and Klionsky, D. J. (2017). Functional impairment in RHOT1/Miro1 degradation and mitophagy is a shared feature in familial and sporadic Parkinson disease. Autophagy 13 (8), 1259–1261. doi:10.1080/15548627.2017.1327512
Latario, C. J., Schoenfeld, L. W., Howarth, C. L., Pickrell, L. E., Begum, F., Fischer, D. A., et al. (2020). Tumor microtubes connect pancreatic cancer cells in an Arp2/3 complex-dependent manner. Mol. Biol. Cell. 31 (12), 1259–1272. doi:10.1091/mbc.E19-11-0605
Lazar, S., and Goldfinger, L. E. (2021). Platelets and extracellular vesicles and their cross talk with cancer. Blood 137 (23), 3192–3200. doi:10.1182/blood.2019004119
Lazarou, M., Sliter, D. A., Kane, L. A., Sarraf, S. A., Wang, C., Burman, J. L., et al. (2015). The ubiquitin kinase PINK1 recruits autophagy receptors to induce mitophagy. Nature 524 (7565), 309–314. doi:10.1038/nature14893
Lee, C. A., Chin, L. S., and Li, L. (2018). Hypertonia-linked protein Trak1 functions with mitofusins to promote mitochondrial tethering and fusion. Protein Cell. 9 (8), 693–716. doi:10.1007/s13238-017-0469-4
Lee, K. S., Huh, S., Lee, S., Wu, Z., Kim, A. K., Kang, H. Y., et al. (2018). Altered ER-mitochondria contact impacts mitochondria calcium homeostasis and contributes to neurodegeneration in vivo in disease models. Proc. Natl. Acad. Sci. U. S. A. 115 (38), E8844–E8853. doi:10.1073/pnas.1721136115
Lee, S., Lee, K. S., Huh, S., Liu, S., Lee, D. Y., Hong, S. H., et al. (2016). Polo kinase phosphorylates Miro to control ER-mitochondria contact sites and mitochondrial Ca(2+) homeostasis in neural stem cell development. Dev. Cell. 37 (2), 174–189. doi:10.1016/j.devcel.2016.03.023
Lee, Y. G., Park, D. H., and Chae, Y. C. (2022). Role of mitochondrial stress response in cancer progression. Cells 11 (5), 771. doi:10.3390/cells11050771
Levoux, J., Prola, A., Lafuste, P., Gervais, M., Chevallier, N., Koumaiha, Z., et al. (2021). Platelets facilitate the wound-healing capability of mesenchymal stem cells by mitochondrial transfer and metabolic reprogramming. Cell. Metab. 33 (3), 688–690. doi:10.1016/j.cmet.2021.02.003
Li, B., Zhang, Y., Li, H., Shen, H., Wang, Y., Li, X., et al. (2020). Miro1 regulates neuronal mitochondrial transport and distribution to alleviate neuronal damage in secondary brain injury after intracerebral hemorrhage in rats. Cell. Mol. Neurobiol. 41, 795–812. published online 2020 June 4. doi:10.1007/s10571-020-00887-2
Li, Q., Yao, L., Wei, Y., Geng, S., He, C., Jiang, H., et al. (2015). Role of RHOT1 on migration and proliferation of pancreatic cancer. Am. J. Cancer Res. 5 (4), 1460–1470.
Li, X., Wang, R., Xun, X., Jiao, W., Zhang, M., Wang, S., et al. (2015). The Rho GTPase family genes in Bivalvia genomes: Sequence, evolution and expression analysis. PLoS One 10 (12), e0143932. doi:10.1371/journal.pone.0143932
Liu, K., Ji, K., Guo, L., Wu, W., Lu, H., Shan, P., et al. (2014). Mesenchymal stem cells rescue injured endothelial cells in an in vitro ischemia-reperfusion model via tunneling nanotube like structure-mediated mitochondrial transfer. Microvasc. Res. 92, 10–18. doi:10.1016/j.mvr.2014.01.008
Ljubojevic, N., Henderson, J. M., and Zurzolo, C. (2021). The ways of actin: Why tunneling nanotubes are unique cell protrusions. Trends Cell. Biol. 31 (2), 130–142. doi:10.1016/j.tcb.2020.11.008
Lopez-Domenech, G., Covill-Cooke, C., Ivankovic, D., Halff, E. F., Sheehan, D. F., Norkett, R., et al. (2018). Miro proteins coordinate microtubule- and actin-dependent mitochondrial transport and distribution. EMBO J. 37 (3), 321–336. doi:10.15252/embj.201696380
Lopez-Domenech, G., Higgs, N. F., Vaccaro, V., Ros, H., Arancibia-Carcamo, I. L., MacAskill, A. F., et al. (2016). Loss of dendritic complexity precedes neurodegeneration in a mouse model with disrupted mitochondrial distribution in mature dendrites. Cell. Rep. 17 (2), 317–327. doi:10.1016/j.celrep.2016.09.004
Lotfi, S., Nasser, H., Noyori, O., Hiyoshi, M., Takeuchi, H., Koyanagi, Y., et al. (2020). M-Sec facilitates intercellular transmission of HIV-1 through multiple mechanisms. Retrovirology 17 (1), 20. doi:10.1186/s12977-020-00528-y
Lou, E., Fujisawa, S., Barlas, A., Romin, Y., Manova-Todorova, K., Moore, M. A., et al. (2012a). Tunneling nanotubes: A new paradigm for studying intercellular communication and therapeutics in cancer. Commun. Integr. Biol. 5 (4), 399–403. doi:10.4161/cib.20569
Lou, E., Fujisawa, S., Morozov, A., Barlas, A., Romin, Y., Dogan, Y., et al. (2012b). Tunneling nanotubes provide a unique conduit for intercellular transfer of cellular contents in human malignant pleural mesothelioma. PLoS One 7 (3), e33093. doi:10.1371/journal.pone.0033093
Lu, J., Zheng, X., Li, F., Yu, Y., Chen, Z., Liu, Z., et al. (2017). Tunneling nanotubes promote intercellular mitochondria transfer followed by increased invasiveness in bladder cancer cells. Oncotarget 8 (9), 15539–15552. doi:10.18632/oncotarget.14695
Luchetti, F., Canonico, B., Arcangeletti, M., Guescini, M., Cesarini, E., Stocchi, V., et al. (2012). Fas signalling promotes intercellular communication in T cells. PLoS One 7 (4), e35766. doi:10.1371/journal.pone.0035766
MacAskill, A. F., Brickley, K., Stephenson, F. A., and Kittler, J. T. (2009a). GTPase dependent recruitment of Grif-1 by Miro1 regulates mitochondrial trafficking in hippocampal neurons. Mol. Cell. Neurosci. 40 (3), 301–312. doi:10.1016/j.mcn.2008.10.016
MacAskill, A. F., and Kittler, J. T. (2010). Control of mitochondrial transport and localization in neurons. Trends Cell. Biol. 20 (2), 102–112. doi:10.1016/j.tcb.2009.11.002
MacAskill, A. F., Rinholm, J. E., Twelvetrees, A. E., Arancibia-Carcamo, I. L., Muir, J., Fransson, A., et al. (2009b). Miro1 is a calcium sensor for glutamate receptor-dependent localization of mitochondria at synapses. Neuron 61 (4), 541–555. doi:10.1016/j.neuron.2009.01.030
Mahrouf-Yorgov, M., Augeul, L., Da Silva, C. C., Jourdan, M., Rigolet, M., Manin, S., et al. (2017). Mesenchymal stem cells sense mitochondria released from damaged cells as danger signals to activate their rescue properties. Cell. Death Differ. 24 (7), 1224–1238. doi:10.1038/cdd.2017.51
Marlein, C. R., Piddock, R. E., Mistry, J. J., Zaitseva, L., Hellmich, C., Horton, R. H., et al. (2019). CD38-Driven mitochondrial trafficking promotes bioenergetic plasticity in multiple myeloma. Cancer Res. 79 (9), 2285–2297. doi:10.1158/0008-5472.CAN-18-0773
Marlein, C. R., Zaitseva, L., Piddock, R. E., Robinson, S. D., Edwards, D. R., Shafat, M. S., et al. (2017). NADPH oxidase-2 derived superoxide drives mitochondrial transfer from bone marrow stromal cells to leukemic blasts. Blood 130 (14), 1649–1660. doi:10.1182/blood-2017-03-772939
Mbefo, M. K., Paleologou, K. E., Boucharaba, A., Oueslati, A., Schell, H., Fournier, M., et al. (2010). Phosphorylation of synucleins by members of the Polo-like kinase family. J. Biol. Chem. 285 (4), 2807–2822. doi:10.1074/jbc.M109.081950
Mills, K. M., Brocardo, M. G., and Henderson, B. R. (2016). APC binds the Miro/Milton motor complex to stimulate transport of mitochondria to the plasma membrane. Mol. Biol. Cell. 27 (3), 466–482. doi:10.1091/mbc.E15-09-0632
Misko, A., Jiang, S., Wegorzewska, I., Milbrandt, J., and Baloh, R. H. (2010). Mitofusin 2 is necessary for transport of axonal mitochondria and interacts with the Miro/Milton complex. J. Neurosci. 30 (12), 4232–4240. doi:10.1523/JNEUROSCI.6248-09.2010
Mittal, R., Karhu, E., Wang, J. S., Delgado, S., Zukerman, R., Mittal, J., et al. (2019). Cell communication by tunneling nanotubes: Implications in disease and therapeutic applications. J. Cell. Physiol. 234 (2), 1130–1146. doi:10.1002/jcp.27072
Mittelbrunn, M., and Sanchez-Madrid, F. (2012). Intercellular communication: Diverse structures for exchange of genetic information. Nat. Rev. Mol. Cell. Biol. 13 (5), 328–335. doi:10.1038/nrm3335
Modi, S., Lopez-Domenech, G., Halff, E. F., Covill-Cooke, C., Ivankovic, D., Melandri, D., et al. (2019). Miro clusters regulate ER-mitochondria contact sites and link cristae organization to the mitochondrial transport machinery. Nat. Commun. 10 (1), 4399. doi:10.1038/s41467-019-12382-4
Moore, D. J., West, A. B., Dikeman, D. A., Dawson, V. L., and Dawson, T. M. (2008). Parkin mediates the degradation-independent ubiquitination of Hsp70. J. Neurochem. 105 (5), 1806–1819. doi:10.1111/j.1471-4159.2008.05261.x
Moschoi, R., Imbert, V., Nebout, M., Chiche, J., Mary, D., Prebet, T., et al. (2016). Protective mitochondrial transfer from bone marrow stromal cells to acute myeloid leukemic cells during chemotherapy. Blood 128 (2), 253–264. doi:10.1182/blood-2015-07-655860
Nahacka, Z., Zobalova, R., Dubisova, M., Rohlena, J., and Neuzil, J. (2021). Miro proteins connect mitochondrial function and intercellular transport. Crit. Rev. Biochem. Mol. Biol. 56 (4), 401–425. doi:10.1080/10409238.2021.1925216
Naik, M. U., Pham, N. T., Beebe, K., Dai, W., and Naik, U. P. (2011). Calcium-dependent inhibition of polo-like kinase 3 activity by CIB1 in breast cancer cells. Int. J. Cancer 128 (3), 587–596. doi:10.1002/ijc.25388
Nguyen, E. K., Koval, O. M., Noble, P., Broadhurst, K., Allamargot, C., Wu, M., et al. (2018). CaMKII (Ca(2+)/calmodulin-dependent kinase II) in mitochondria of smooth muscle cells controls mitochondrial mobility, migration, and neointima formation. Arterioscler. Thromb. Vasc. Biol. 38 (6), 1333–1345. doi:10.1161/ATVBAHA.118.310951
Nguyen, T. T., Oh, S. S., Weaver, D., Lewandowska, A., Maxfield, D., Schuler, M. H., et al. (2014). Loss of Miro1-directed mitochondrial movement results in a novel murine model for neuron disease. Proc. Natl. Acad. Sci. U. S. A. 111 (35), E3631–E3640. doi:10.1073/pnas.1402449111
Nicolas-Avila, J. A., Lechuga-Vieco, A. V., Esteban-Martinez, L., Sanchez-Diaz, M., Diaz-Garcia, E., Santiago, D. J., et al. (2020). A network of macrophages supports mitochondrial homeostasis in the heart. Cell. 183 (1), 94–109. e123. doi:10.1016/j.cell.2020.08.031
Niescier, R. F., Chang, K. T., and Min, K. T. (2013). Miro, MCU, and calcium: Bridging our understanding of mitochondrial movement in axons. Front. Cell. Neurosci. 7, 148. doi:10.3389/fncel.2013.00148
Niescier, R. F., Hong, K., Park, D., and Min, K. T. (2018). MCU interacts with Miro1 to modulate mitochondrial functions in neurons. J. Neurosci. 38 (20), 4666–4677. doi:10.1523/JNEUROSCI.0504-18.2018
Oeding, S. J., Majstrowicz, K., Hu, X. P., Schwarz, V., Freitag, A., Honnert, U., et al. (2018). Identification of Miro1 and Miro2 as mitochondrial receptors for myosin XIX. J. Cell. Sci. 131 (17), jcs219469. doi:10.1242/jcs.219469
Okumoto, K., Ono, T., Toyama, R., Shimomura, A., Nagata, A., Fujiki, Y., et al. (2018). New splicing variants of mitochondrial Rho GTPase-1 (Miro1) transport peroxisomes. J. Cell. Biol. 217 (2), 619–633. doi:10.1083/jcb.201708122
Olzmann, J. A., Li, L., Chudaev, M. V., Chen, J., Perez, F. A., Palmiter, R. D., et al. (2007). Parkin-mediated K63-linked polyubiquitination targets misfolded DJ-1 to aggresomes via binding to HDAC6. J. Cell. Biol. 178 (6), 1025–1038. doi:10.1083/jcb.200611128
Osswald, M., Jung, E., Sahm, F., Solecki, G., Venkataramani, V., Blaes, J., et al. (2015). Brain tumour cells interconnect to a functional and resistant network. Nature 528 (7580), 93–98. doi:10.1038/nature16071
Paliwal, S., Chaudhuri, R., Agrawal, A., and Mohanty, S. (2018). Regenerative abilities of mesenchymal stem cells through mitochondrial transfer. J. Biomed. Sci. 25 (1), 31. doi:10.1186/s12929-018-0429-1
Panchal, K., and Tiwari, A. K. (2021). Miro (Mitochondrial Rho GTPase), a key player of mitochondrial axonal transport and mitochondrial dynamics in neurodegenerative diseases. Mitochondrion 56, 118–135. doi:10.1016/j.mito.2020.10.005
Park, G. H., Park, J. H., and Chung, K. C. (2021). Precise control of mitophagy through ubiquitin proteasome system and deubiquitin proteases and their dysfunction in Parkinson's disease. BMB Rep. 54 (12), 592–600. doi:10.5483/bmbrep.2021.54.12.107
Park, S., Foote, P. K., Krist, D. T., Rice, S. E., and Statsyuk, A. V. (2017). UbMES and UbFluor: Novel probes for ring-between-ring (RBR) E3 ubiquitin ligase PARKIN. J. Biol. Chem. 292 (40), 16539–16553. doi:10.1074/jbc.M116.773200
Pasquier, J., Guerrouahen, B. S., Al Thawadi, H., Ghiabi, P., Maleki, M., Abu-Kaoud, N., et al. (2013). Preferential transfer of mitochondria from endothelial to cancer cells through tunneling nanotubes modulates chemoresistance. J. Transl. Med. 11, 94. doi:10.1186/1479-5876-11-94
Patergnani, S., Danese, A., Bouhamida, E., Aguiari, G., Previati, M., Pinton, P., et al. (2020). Various aspects of calcium signaling in the regulation of apoptosis, autophagy, cell proliferation, and cancer. Int. J. Mol. Sci. 21 (21), E8323. doi:10.3390/ijms21218323
Pavlova, N. N., Zhu, J., and Thompson, C. B. (2022). The hallmarks of cancer metabolism: Still emerging. Cell. Metab. 34 (3), 355–377. doi:10.1016/j.cmet.2022.01.007
Peters, D. T., Kay, L., Eswaran, J., Lakey, J. H., and Soundararajan, M. (2018). Human Miro proteins act as NTP hydrolases through a novel, non-canonical catalytic mechanism. Int. J. Mol. Sci. 19 (12), 3839. doi:10.3390/ijms19123839
Pickles, S., Vigie, P., and Youle, R. J. (2018). Mitophagy and quality control mechanisms in mitochondrial maintenance. Curr. Biol. 28 (4), R170–R185. doi:10.1016/j.cub.2018.01.004
Pinto, G., Brou, C., and Zurzolo, C. (2020). Tunneling nanotubes: The fuel of tumor progression? Trends Cancer 6 (10), 874–888. doi:10.1016/j.trecan.2020.04.012
Pinto, G., Saenz-de-Santa-Maria, I., Chastagner, P., Perthame, E., Delmas, C., Toulas, C., et al. (2021). Patient-derived glioblastoma stem cells transfer mitochondria through tunneling nanotubes in tumor organoids. Biochem. J. 478 (1), 21–39. doi:10.1042/BCJ20200710
Plotnikov, E. Y., Khryapenkova, T. G., Galkina, S. I., Sukhikh, G. T., and Zorov, D. B. (2010). Cytoplasm and organelle transfer between mesenchymal multipotent stromal cells and renal tubular cells in co-culture. Exp. Cell. Res. 316 (15), 2447–2455. doi:10.1016/j.yexcr.2010.06.009
Polak, R., de Rooij, B., Pieters, R., and den Boer, M. L. (2015). B-cell precursor acute lymphoblastic leukemia cells use tunneling nanotubes to orchestrate their microenvironment. Blood 126 (21), 2404–2414. doi:10.1182/blood-2015-03-634238
Porporato, P. E., Filigheddu, N., Pedro, J. M. B., Kroemer, G., and Galluzzi, L. (2018). Mitochondrial metabolism and cancer. Cell. Res. 28 (3), 265–280. doi:10.1038/cr.2017.155
Qin, Y., Jiang, X., Yang, Q., Zhao, J., Zhou, Q., Zhou, Y., et al. (2021). The functions, methods, and mobility of mitochondrial transfer between cells. Front. Oncol. 11, 672781. doi:10.3389/fonc.2021.672781
Qu, S., Hao, X., Song, W., Niu, K., Yang, X., Zhang, X., et al. (2019). Circular RNA circRHOT1 is upregulated and promotes cell proliferation and invasion in pancreatic cancer. Epigenomics 11 (1), 53–63. doi:10.2217/epi-2018-0051
Quintero, O. A., DiVito, M. M., Adikes, R. C., Kortan, M. B., Case, L. B., Lier, A. J., et al. (2009). Human Myo19 is a novel myosin that associates with mitochondria. Curr. Biol. 19 (23), 2008–2013. doi:10.1016/j.cub.2009.10.026
Raghavan, A., Rao, P., Neuzil, J., Pountney, D. L., and Nath, S. (2021). Oxidative stress and Rho GTPases in the biogenesis of tunnelling nanotubes: Implications in disease and therapy. Cell. Mol. Life Sci. 79 (1), 36. doi:10.1007/s00018-021-04040-0
Ranzinger, J., Rustom, A., and Schwenger, V. (2014). Membrane nanotubes between peritoneal mesothelial cells: Functional connectivity and crucial participation during inflammatory reactions. Front. Physiol. 5, 412. doi:10.3389/fphys.2014.00412
Reis, K., Fransson, A., and Aspenstrom, P. (2009). The Miro GTPases: At the heart of the mitochondrial transport machinery. FEBS Lett. 583 (9), 1391–1398. doi:10.1016/j.febslet.2009.04.015
Resnik, N., Prezelj, T., De Luca, G. M. R., Manders, E., Polishchuk, R., Veranic, P., et al. (2018). Helical organization of microtubules occurs in a minority of tunneling membrane nanotubes in normal and cancer urothelial cells. Sci. Rep. 8 (1), 17133. doi:10.1038/s41598-018-35370-y
Richard, T. J. C., Herzog, L. K., Vornberger, J., Rahmanto, A. S., Sangfelt, O., Salomons, F. A., et al. (2020). K63-linked ubiquitylation induces global sequestration of mitochondria. Sci. Rep. 10 (1), 22334. doi:10.1038/s41598-020-78845-7
Rieusset, J. (2017). Role of endoplasmic reticulum-mitochondria communication in Type 2 diabetes. Adv. Exp. Med. Biol. 997, 171–186. doi:10.1007/978-981-10-4567-7_13
Rivadeneira, D. B., Caino, M. C., Seo, J. H., Angelin, A., Wallace, D. C., Languino, L. R., et al. (2015). Survivin promotes oxidative phosphorylation, subcellular mitochondrial repositioning, and tumor cell invasion. Sci. Signal. 8 (389), ra80. doi:10.1126/scisignal.aab1624
Rizzuto, R., Pinton, P., Carrington, W., Fay, F. S., Fogarty, K. E., Lifshitz, L. M., et al. (1998). Close contacts with the endoplasmic reticulum as determinants of mitochondrial Ca2+ responses. Science 280 (5370), 1763–1766. doi:10.1126/science.280.5370.1763
Roehlecke, C., and Schmidt, M. H. H. (2020). Tunneling nanotubes and tumor microtubes in cancer. Cancers (Basel) 12 (4), E857. doi:10.3390/cancers12040857
Romero-Garcia, S., and Prado-Garcia, H. (2019). Mitochondrial calcium: Transport and modulation of cellular processes in homeostasis and cancer (Review). Int. J. Oncol. 54 (4), 1155–1167. doi:10.3892/ijo.2019.4696
Rustom, A., Saffrich, R., Markovic, I., Walther, P., and Gerdes, H. H. (2004). Nanotubular highways for intercellular organelle transport. Science 303 (5660), 1007–1010. doi:10.1126/science.1093133
Rustom, A. (2016). The missing link: Does tunnelling nanotube-based supercellularity provide a new understanding of chronic and lifestyle diseases? Open Biol. 6 (6), 160057. doi:10.1098/rsob.160057
Saeed, M. (2018). Genomic convergence of locus-based GWAS meta-analysis identifies AXIN1 as a novel Parkinson's gene. Immunogenetics 70 (9), 563–570. doi:10.1007/s00251-018-1068-0
Safiulina, D., Kuum, M., Choubey, V., Gogichaishvili, N., Liiv, J., Hickey, M. A., et al. (2019a). Miro proteins prime mitochondria for Parkin translocation and mitophagy. EMBO J. 38 (2), e99384. doi:10.15252/embj.201899384
Safiulina, D., Kuum, M., Choubey, V., Hickey, M. A., and Kaasik, A. (2019b). Mitochondrial transport proteins RHOT1 and RHOT2 serve as docking sites for PRKN-mediated mitophagy. Autophagy 15 (5), 930–931. doi:10.1080/15548627.2019.1586260
Saha, T., Dash, C., Jayabalan, R., Khiste, S., Kulkarni, A., Kurmi, K., et al. (2022). Intercellular nanotubes mediate mitochondrial trafficking between cancer and immune cells. Nat. Nanotechnol. 17 (1), 98–106. doi:10.1038/s41565-021-01000-4
Sahinbegovic, H., Jelinek, T., Hrdinka, M., Bago, J. R., Turi, M., Sevcikova, T., et al. (2020). Intercellular mitochondrial transfer in the tumor microenvironment. Cancers (Basel) 12 (7), E1787. doi:10.3390/cancers12071787
Sahu, P., Jena, S. R., and Samanta, L. (2018). Tunneling nanotubes: A versatile target for cancer therapy. Curr. Cancer Drug Targets 18 (6), 514–521. doi:10.2174/1568009618666171129222637
Sala-Vila, A., Navarro-Lerida, I., Sanchez-Alvarez, M., Bosch, M., Calvo, C., Lopez, J. A., et al. (2016). Interplay between hepatic mitochondria-associated membranes, lipid metabolism and caveolin-1 in mice. Sci. Rep. 6, 27351. doi:10.1038/srep27351
Salaud, C., Alvarez-Arenas, A., Geraldo, F., Belmonte-Beitia, J., Calvo, G. F., Gratas, C., et al. (2020). Mitochondria transfer from tumor-activated stromal cells (TASC) to primary Glioblastoma cells. Biochem. Biophys. Res. Commun. 533 (1), 139–147. doi:10.1016/j.bbrc.2020.08.101
Saotome, M., Safiulina, D., Szabadkai, G., Das, S., Fransson, A., Aspenstrom, P., et al. (2008). Bidirectional Ca2+-dependent control of mitochondrial dynamics by the Miro GTPase. Proc. Natl. Acad. Sci. U. S. A. 105 (52), 20728–20733. doi:10.1073/pnas.0808953105
Sáenz-de-Santa-María, I., Bernardo-Castiñeira, C., Enciso, E., García-Moreno, I., Chiara, J. L., Suarez, C., et al. (2017). Control of long-distance cell-to-cell communication and autophagosome transfer in squamous cell carcinoma via tunneling nanotubes. Oncotarget. 8 (13), 20939–20960. doi:10.18632/oncotarget.15467
Sartori-Rupp, A., Cordero Cervantes, D., Pepe, A., Gousset, K., Delage, E., Corroyer-Dulmont, S., et al. (2019). Correlative cryo-electron microscopy reveals the structure of TNTs in neuronal cells. Nat. Commun. 10 (1), 342. doi:10.1038/s41467-018-08178-7
Schiller, C., Diakopoulos, K. N., Rohwedder, I., Kremmer, E., von Toerne, C., Ueffing, M., et al. (2013). LST1 promotes the assembly of a molecular machinery responsible for tunneling nanotube formation. J. Cell. Sci. 126 (Pt 3), 767–777. doi:10.1242/jcs.114033
Schuler, M. H., Lewandowska, A., Caprio, G. D., Skillern, W., Upadhyayula, S., Kirchhausen, T., et al. (2017). Miro1-mediated mitochondrial positioning shapes intracellular energy gradients required for cell migration. Mol. Biol. Cell. 28 (16), 2159–2169. doi:10.1091/mbc.E16-10-0741
Schwarz, T. L. (2013). Mitochondrial trafficking in neurons. Cold Spring Harb. Perspect. Biol. 5 (6), a011304. doi:10.1101/cshperspect.a011304
Senft, D., and Ronai, Z. A. (2016). Regulators of mitochondrial dynamics in cancer. Curr. Opin. Cell. Biol. 39, 43–52. doi:10.1016/j.ceb.2016.02.001
Seo, J. H., Agarwal, E., Bryant, K. G., Caino, M. C., Kim, E. T., Kossenkov, A. V., et al. (2018). Syntaphilin ubiquitination regulates mitochondrial dynamics and tumor cell movements. Cancer Res. 78 (15), 4215–4228. doi:10.1158/0008-5472.CAN-18-0595
Shan, Z., Fa, W. H., Tian, C. R., Yuan, C. S., and Jie, N. (2022). Mitophagy and mitochondrial dynamics in type 2 diabetes mellitus treatment. Aging (Albany NY) 14 (6), 2902–2919. doi:10.18632/aging.203969
Shanmughapriya, S., Langford, D., and Natarajaseenivasan, K. (2020). Inter and Intracellular mitochondrial trafficking in health and disease. Ageing Res. Rev. 62, 101128. doi:10.1016/j.arr.2020.101128
Shlevkov, E., Kramer, T., Schapansky, J., LaVoie, M. J., and Schwarz, T. L. (2016). Miro phosphorylation sites regulate Parkin recruitment and mitochondrial motility. Proc. Natl. Acad. Sci. U. S. A. 113 (41), E6097–E6106. doi:10.1073/pnas.1612283113
Simmen, T., Aslan, J. E., Blagoveshchenskaya, A. D., Thomas, L., Wan, L., Xiang, Y., et al. (2005). PACS-2 controls endoplasmic reticulum-mitochondria communication and Bid-mediated apoptosis. EMBO J. 24 (4), 717–729. doi:10.1038/sj.emboj.7600559
Sinha, P., Islam, M. N., Bhattacharya, S., and Bhattacharya, J. (2016). Intercellular mitochondrial transfer: Bioenergetic crosstalk between cells. Curr. Opin. Genet. Dev. 38, 97–101. doi:10.1016/j.gde.2016.05.002
Smith, K. P., Focia, P. J., Chakravarthy, S., Landahl, E. C., Klosowiak, J. L., Rice, S. E., et al. (2020). Insight into human Miro1/2 domain organization based on the structure of its N-terminal GTPase. J. Struct. Biol. 212 (3), 107656. doi:10.1016/j.jsb.2020.107656
Soubannier, V., McLelland, G. L., Zunino, R., Braschi, E., Rippstein, P., Fon, E. A., et al. (2012). A vesicular transport pathway shuttles cargo from mitochondria to lysosomes. Curr. Biol. 22 (2), 135–141. doi:10.1016/j.cub.2011.11.057
Stephan, T., Bruser, C., Deckers, M., Steyer, A. M., Balzarotti, F., Barbot, M., et al. (2020). MICOS assembly controls mitochondrial inner membrane remodeling and crista junction redistribution to mediate cristae formation. EMBO J. 39 (14), e104105. doi:10.15252/embj.2019104105
Stephen, T. L., Higgs, N. F., Sheehan, D. F., Al Awabdh, S., Lopez-Domenech, G., Arancibia-Carcamo, I. L., et al. (2015). Miro1 regulates activity-driven positioning of mitochondria within astrocytic processes apposed to synapses to regulate intracellular calcium signaling. J. Neurosci. 35 (48), 15996–16011. doi:10.1523/JNEUROSCI.2068-15.2015
Sugiura, A., Mattie, S., Prudent, J., and McBride, H. M. (2017). Newly born peroxisomes are a hybrid of mitochondrial and ER-derived pre-peroxisomes. Nature 542 (7640), 251–254. doi:10.1038/nature21375
Sun, Y., Vashisht, A. A., Tchieu, J., Wohlschlegel, J. A., and Dreier, L. (2012). Voltage-dependent anion channels (VDACs) recruit Parkin to defective mitochondria to promote mitochondrial autophagy. J. Biol. Chem. 287 (48), 40652–40660. doi:10.1074/jbc.M112.419721
Tan, A. S., Baty, J. W., Dong, L. F., Bezawork-Geleta, A., Endaya, B., Goodwin, J., et al. (2015). Mitochondrial genome acquisition restores respiratory function and tumorigenic potential of cancer cells without mitochondrial DNA. Cell. Metab. 21 (1), 81–94. doi:10.1016/j.cmet.2014.12.003
Tang, Z., Li, C., Kang, B., Gao, G., Li, C., Zhang, Z., et al. (2017). Gepia: A web server for cancer and normal gene expression profiling and interactive analyses. Nucleic Acids Res. 45 (W1), W98–W102. doi:10.1093/nar/gkx247
Tasca, A., Astleford, K., Lederman, A., Jensen, E. D., Lee, B. S., Gopalakrishnan, R., et al. (2017). Regulation of osteoclast differentiation by myosin X. Sci. Rep. 7 (1), 7603. doi:10.1038/s41598-017-07855-9
Thayanithy, V., Dickson, E. L., Steer, C., Subramanian, S., and Lou, E. (2014). Tumor-stromal cross talk: Direct cell-to-cell transfer of oncogenic microRNAs via tunneling nanotubes. Transl. Res. 164 (5), 359–365. doi:10.1016/j.trsl.2014.05.011
Tiwari, V., Koganti, R., Russell, G., Sharma, A., and Shukla, D. (2021). Role of tunneling nanotubes in viral infection, neurodegenerative disease, and cancer. Front. Immunol. 12, 680891. doi:10.3389/fimmu.2021.680891
Torralba, D., Baixauli, F., and Sanchez-Madrid, F. (2016). Mitochondria know No boundaries: Mechanisms and functions of intercellular mitochondrial transfer. Front. Cell. Dev. Biol. 4, 107. doi:10.3389/fcell.2016.00107
Tsai, P. I., Papakyrikos, A. M., Hsieh, C. H., and Wang, X. (2017). Drosophila MIC60/mitofilin conducts dual roles in mitochondrial motility and crista structure. Mol. Biol. Cell. 28 (24), 3471–3479. doi:10.1091/mbc.E17-03-0177
Tseng, N., Lambie, S. C., Huynh, C. Q., Sanford, B., Patel, M., Herson, P. S., et al. (2021). Mitochondrial transfer from mesenchymal stem cells improves neuronal metabolism after oxidant injury in vitro: The role of Miro1. J. Cereb. Blood Flow. Metab. 41 (4), 761–770. doi:10.1177/0271678X20928147
Tubbs, E., Theurey, P., Vial, G., Bendridi, N., Bravard, A., Chauvin, M. A., et al. (2014). Mitochondria-associated endoplasmic reticulum membrane (MAM) integrity is required for insulin signaling and is implicated in hepatic insulin resistance. Diabetes 63 (10), 3279–3294. doi:10.2337/db13-1751
UniProt Consortium (2019). UniProt: A worldwide hub of protein knowledge. Nucleic Acids Res. 47 (D1), D506–D515. doi:10.1093/nar/gky1049
Valenti, D., Vacca, R. A., Moro, L., and Atlante, A. (2021). Mitochondria can cross cell boundaries: An overview of the biological relevance, pathophysiological implications and therapeutic perspectives of intercellular mitochondrial transfer. Int. J. Mol. Sci. 22 (15), 8312. doi:10.3390/ijms22158312
Vallabhaneni, K. C., Haller, H., and Dumler, I. (2012). Vascular smooth muscle cells initiate proliferation of mesenchymal stem cells by mitochondrial transfer via tunneling nanotubes. Stem Cells Dev. 21 (17), 3104–3113. doi:10.1089/scd.2011.0691
Vance, J. E. (1990). Phospholipid synthesis in a membrane fraction associated with mitochondria. J. Biol. Chem. 265 (13), 7248–7256. doi:10.1016/S0021-9258(19)39106-9
Vander Heiden, M. G., Cantley, L. C., and Thompson, C. B. (2009). Understanding the warburg effect: The metabolic requirements of cell proliferation. Science 324 (5930), 1029–1033. doi:10.1126/science.1160809
Vaupel, P., Schmidberger, H., and Mayer, A. (2019). The warburg effect: Essential part of metabolic reprogramming and central contributor to cancer progression. Int. J. Radiat. Biol. 95 (7), 912–919. doi:10.1080/09553002.2019.1589653
Vignais, M. L., Caicedo, A., Brondello, J. M., and Jorgensen, C. (2017). Cell connections by tunneling nanotubes: Effects of mitochondrial trafficking on target cell metabolism, homeostasis, and response to therapy. Stem Cells Int. 2017, 6917941. doi:10.1155/2017/6917941
Vona, R., Mileo, A. M., and Matarrese, P. (2021). Microtubule-based mitochondrial dynamics as a valuable therapeutic target in cancer. Cancers (Basel) 13 (22), 5812. doi:10.3390/cancers13225812
Wan, B., LaNoue, K. F., Cheung, J. Y., and Scaduto, R. C. (1989). Regulation of citric acid cycle by calcium. J. Biol. Chem. 264 (23), 13430–13439. doi:10.1016/s0021-9258(18)80015-1
Wang, F., Chen, X., Cheng, H., Song, L., Liu, J., Caplan, S., et al. (2021). MICAL2PV suppresses the formation of tunneling nanotubes and modulates mitochondrial trafficking. EMBO Rep. 22 (7), e52006. doi:10.15252/embr.202052006
Wang, J., Liu, X., Qiu, Y., Shi, Y., Cai, J., Wang, B., et al. (2018). Cell adhesion-mediated mitochondria transfer contributes to mesenchymal stem cell-induced chemoresistance on T cell acute lymphoblastic leukemia cells. J. Hematol. Oncol. 11 (1), 11. doi:10.1186/s13045-018-0554-z
Wang, L., Long, H., Zheng, Q., Bo, X., Xiao, X., Li, B., et al. (2019). Circular RNA circRHOT1 promotes hepatocellular carcinoma progression by initiation of NR2F6 expression. Mol. Cancer 18 (1), 119. doi:10.1186/s12943-019-1046-7
Wang, X., and Gerdes, H. H. (2015). Transfer of mitochondria via tunneling nanotubes rescues apoptotic PC12 cells. Cell. Death Differ. 22 (7), 1181–1191. doi:10.1038/cdd.2014.211
Wang, X., Winter, D., Ashrafi, G., Schlehe, J., Wong, Y. L., Selkoe, D., et al. (2011). PINK1 and Parkin target Miro for phosphorylation and degradation to arrest mitochondrial motility. Cell. 147 (4), 893–906. doi:10.1016/j.cell.2011.10.018
Wang, Y., Cui, J., Sun, X., and Zhang, Y. (2011). Tunneling-nanotube development in astrocytes depends on p53 activation. Cell. Death Differ. 18 (4), 732–742. doi:10.1038/cdd.2010.147
Warburg, O. (1956). On the origin of cancer cells. Science 123 (3191), 309–314. doi:10.1126/science.123.3191.309
Wennerberg, K., and Der, C. J. (2004). Rho-family GTPases: it's not only rac and Rho (and I like it). J. Cell. Sci. 117 (Pt 8), 1301–1312. doi:10.1242/jcs.01118
Weston, L. J., Stackhouse, T. L., Spinelli, K. J., Boutros, S. W., Rose, E. P., Osterberg, V. R., et al. (2021). Genetic deletion of Polo-like kinase 2 reduces alpha-synuclein serine-129 phosphorylation in presynaptic terminals but not Lewy bodies. J. Biol. Chem. 296, 100273. doi:10.1016/j.jbc.2021.100273
White, R. R., Lin, C., Leaves, I., Castro, I. G., Metz, J., Bateman, B. C., et al. (2020). Miro2 tethers the ER to mitochondria to promote mitochondrial fusion in tobacco leaf epidermal cells. Commun. Biol. 3 (1), 161. doi:10.1038/s42003-020-0872-x
Wittig, D., Wang, X., Walter, C., Gerdes, H. H., Funk, R. H., Roehlecke, C., et al. (2012). Multi-level communication of human retinal pigment epithelial cells via tunneling nanotubes. PLoS One 7 (3), e33195. doi:10.1371/journal.pone.0033195
Yamaoka, S., Nakajima, M., Fujimoto, M., and Tsutsumi, N. (2011). MIRO1 influences the morphology and intracellular distribution of mitochondria during embryonic cell division in Arabidopsis. Plant Cell. Rep. 30 (2), 239–244. doi:10.1007/s00299-010-0926-5
Yang, H., Borg, T. K., Ma, Z., Xu, M., Wetzel, G., Saraf, L. V., et al. (2016). Biochip-based study of unidirectional mitochondrial transfer from stem cells to myocytes via tunneling nanotubes. Biofabrication 8 (1), 015012. doi:10.1088/1758-5090/8/1/015012
Yang, M., Li, C., Yang, S., Xiao, Y., Xiong, X., Chen, W., et al. (2020). Mitochondria-associated ER membranes - the origin site of autophagy. Front. Cell. Dev. Biol. 8, 595. doi:10.3389/fcell.2020.00595
Yang, Y., Ye, G., Zhang, Y. L., He, H. W., Yu, B. Q., Hong, Y. M., et al. (2020). Transfer of mitochondria from mesenchymal stem cells derived from induced pluripotent stem cells attenuates hypoxia-ischemia-induced mitochondrial dysfunction in PC12 cells. Neural Regen. Res. 15 (3), 464–472. doi:10.4103/1673-5374.266058
Yi, M., Weaver, D., and Hajnoczky, G. (2004). Control of mitochondrial motility and distribution by the calcium signal: A homeostatic circuit. J. Cell. Biol. 167 (4), 661–672. doi:10.1083/jcb.200406038
Zampieri, L. X., Silva-Almeida, C., Rondeau, J. D., and Sonveaux, P. (2021). Mitochondrial transfer in cancer: A comprehensive review. Int. J. Mol. Sci. 22 (6), 3245. doi:10.3390/ijms22063245
Zhang, L., Dai, L., and Li, D. (2021). Mitophagy in neurological disorders. J. Neuroinflammation 18 (1), 297. doi:10.1186/s12974-021-02334-5
Zhang, L., and Zhang, Y. (2015). Tunneling nanotubes between rat primary astrocytes and C6 glioma cells alter proliferation potential of glioma cells. Neurosci. Bull. 31 (3), 371–378. doi:10.1007/s12264-014-1522-4
Zhang, Y., Yu, Z., Jiang, D., Liang, X., Liao, S., Zhang, Z., et al. (2016). iPSC-MSCs with high intrinsic MIRO1 and sensitivity to TNF-alpha yield efficacious mitochondrial transfer to rescue anthracycline-induced cardiomyopathy. Stem Cell. Rep. 7 (4), 749–763. doi:10.1016/j.stemcr.2016.08.009
Zhao, J., Zhang, J., Yu, M., Xie, Y., Huang, Y., Wolff, D. W., et al. (2013). Mitochondrial dynamics regulates migration and invasion of breast cancer cells. Oncogene 32 (40), 4814–4824. doi:10.1038/onc.2012.494
Zhao, Y., Song, E., Wang, W., Hsieh, C. H., Wang, X., Feng, W., et al. (2021). Metaxins are core components of mitochondrial transport adaptor complexes. Nat. Commun. 12 (1), 83. doi:10.1038/s41467-020-20346-2
Keywords: cancer, mitochondria, intercellular transfer, Miro, respiration, migration, metastasis
Citation: Nahacka Z, Novak J, Zobalova R and Neuzil J (2022) Miro proteins and their role in mitochondrial transfer in cancer and beyond. Front. Cell Dev. Biol. 10:937753. doi: 10.3389/fcell.2022.937753
Received: 06 May 2022; Accepted: 04 July 2022;
Published: 25 July 2022.
Edited by:
Yi Zhang, First Affiliated Hospital of Zhengzhou University, ChinaReviewed by:
Shrivani Pirahas, University of Calgary, CanadaAkshay Narkar, United States Food and Drug Administration, United States
Copyright © 2022 Nahacka, Novak, Zobalova and Neuzil. This is an open-access article distributed under the terms of the Creative Commons Attribution License (CC BY). The use, distribution or reproduction in other forums is permitted, provided the original author(s) and the copyright owner(s) are credited and that the original publication in this journal is cited, in accordance with accepted academic practice. No use, distribution or reproduction is permitted which does not comply with these terms.
*Correspondence: Zuzana Nahacka, zuzana.nahacka@ibt.cas.cz; Jiri Neuzil, j.neuzil@griffith.edu.au
†These authors have contributed equally to this work and share first authorship