Formation and Developmental Specification of the Odontogenic and Osteogenic Mesenchymes
- 1Laboratory of Odontogenesis and Osteogenesis, Institute of Animal Physiology and Genetics, Academy of Sciences, Brno, Czechia
- 2Department of Histology and Embryology, Third Faculty of Medicine, Charles University, Prague, Czechia
- 3Department of Physiology, University of Veterinary and Pharmaceutical Sciences, Brno, Czechia
Within the mandible, the odontogenic and osteogenic mesenchymes develop in a close proximity and form at about the same time. They both originate from the cranial neural crest. These two condensing ecto-mesenchymes are soon separated from each other by a very loose interstitial mesenchyme, whose cells do not express markers suggesting a neural crest origin. The two condensations give rise to mineralized tissues while the loose interstitial mesenchyme, remains as a soft tissue. This is crucial for proper anchorage of mammalian teeth. The situation in all three regions of the mesenchyme was compared with regard to cell heterogeneity. As the development progresses, the early phenotypic differences and the complexity in cell heterogeneity increases. The differences reported here and their evolution during development progressively specifies each of the three compartments. The aim of this review was to discuss the mechanisms underlying condensation in both the odontogenic and osteogenic compartments as well as the progressive differentiation of all three mesenchymes during development. Very early, they show physical and structural differences including cell density, shape and organization as well as the secretion of three distinct matrices, two of which will mineralize. Based on these data, this review highlights the consecutive differences in cell-cell and cell-matrix interactions, which support the cohesion as well as mechanosensing and mechanotransduction. These are involved in the conversion of mechanical energy into biochemical signals, cytoskeletal rearrangements cell differentiation, or collective cell behavior.
Introduction
During development, epithelially derived organs (e.g., skin appendages, teeth) form from an initial epithelial bud surrounded by a condensed mass of embryonic connective tissue (mesenchyme). The mesenchyme-derived organs (e.g., bones, cartilages) develop from mesenchymal cell condensations (Hall and Miyake, 2000; Giffin et al., 2019).
The odontogenic and osteogenic jaw ecto-mesenchymes originate from cranial neural crest-derived cells (NCDCs). Their condensation takes place in two distinct regions, at about the same time in the embryonic mouse lower jaw. They later diversify into multiple cell types, along with their progressive dental or bone specification (Ruch et al., 1995; Santagati and Rijli, 2003). Cranial neural crest cells are multipotent and differ according to distinct migratory streams and environments at a post-migratory stage (Yuan and Chai, 2019). Both the odontogenic and osteogenic condensations are progressively invaded by exogenous cells, which increases cell diversity. The cellular heterogeneity of the mesenchyme will be discussed below, as well as the mechanisms suggested to be involved in mesenchymal condensation, the progressive specification of the different mesenchymal areas, and the biological interfaces between soft and hard tissues.
The cell diversity in the three mesenchymal areas, including the participation of exogenous cells, is still under investigation in several laboratories. Comparison between situation in all three regions was discussed with regard to their ability to condense and mineralize or not. Particular attention was given to differential cell–cell and cell–matrix interactions and thus mechanosensing and mechanotransduction. All together, the sequential steps and mechanisms involved in it ultimately lead to the formation of a functional unit: a tooth firmly attached to the surrounding mandibular/alveolar bone by means of the non-mineralized periodontal ligament (PDL). Most of the data discussed here are related to the mouse first lower molar, a very common model. However, the general aspects considered here should be applicable to other models.
Mesenchymal Cell Heterogeneity in the Mandible
In the mouse mandible at embryonic day (ED)12.5, two distant condensations are separated from each other by a looser uncondensed mesenchyme: an osteogenic one, deep in the embryonic jaw and the odontogenic one, adjacent to the thickened dental epithelium/dental lamina in the prospective first molar (M1) region (Figures 1A–C). At early stages, the external limits of the two condensations can hardly be determined since there is a continuous decreasing gradient in cell density toward the surrounding uncondensed mesenchyme (Figures 1A,B). Both the odontogenic mesenchymal condensation and the dental epithelium represent the ecto-mesenchymal and epithelial components of a developing tooth primordium, respectively.
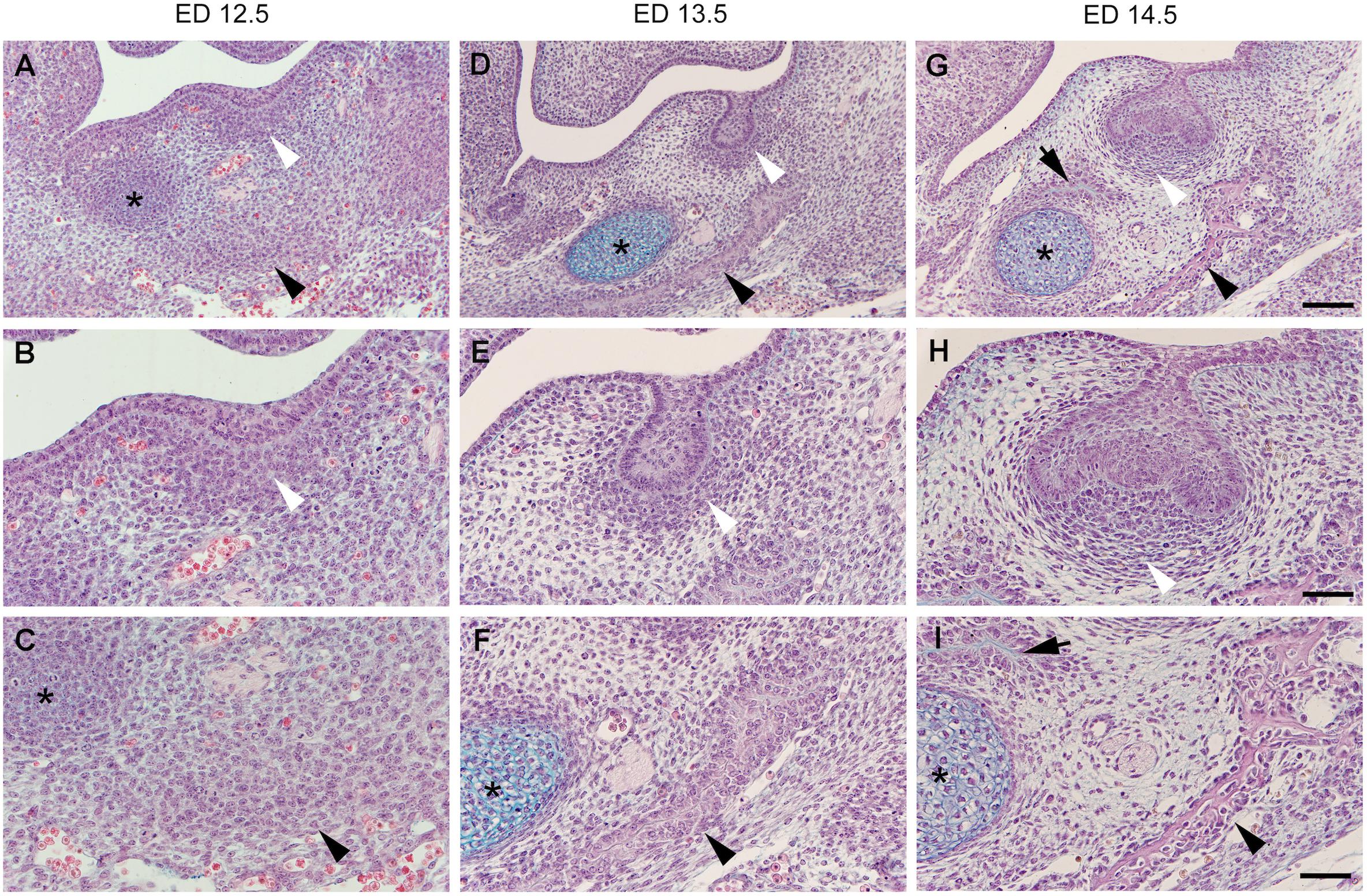
Figure 1. Histological sections of the embryonic mouse lower jaw at the level of M1. The two odontogenic (white arrowhead) and osteogenic (black arrowhead) mesenchymal condensations are distant from each other being separated by a looser interstitial mesenchyme at embryonic day (ED)12.5 (A–C). Jaw bone appears at ED13.5 (D–F), and ED14.5 (G–I), and its development is accelerated on the lateral side (black arrowhead) when compared to the medial side (black arrow). Both the bone and tooth germ grow and approach each other at the expense of the area of the interstitial mesenchyme. The dental follicle cells achieve progressively a typical pattern (compare to Figure 2). Although mineralization will take place in both osteogenic and odontogenic condensations, the interstitial mesenchyme (see Figure 2) will never mineralize in normal conditions. * Refers to the Meckel’s cartilage, Bars = 100 mm (A,D,G) and 50 mm (B,C,E,F,H,I).
At ED13.5, the odontogenic condensation becomes more pronounced and surrounds the dental epithelium, which acquires the shape of a tooth bud (Figures 1D,E). A bone matrix is already visible in the osteogenic condensation in the mouse lower jaw (Figure 1F). At early stages, the intramembranous bone formation is characterized by apposition only. The characteristic of intramembranous bone formation is the absence of a chondrogenic step as it exists during endochondral bone development. Progenitor cells present in the condensation differentiate directly into osteoblasts (Berendsen and Olsen, 2015). Signaling and transcriptional regulation, involved in osteoblasts differentiation, have been investigated (for review, see Huang et al., 2007; Ferguson and Atit, 2019).
Within 2 days, from ED13 to ED15, when cell differentiation occurs, the osteogenic condensation turns into a vascularized bone containing bone specific cells: (e.g., Alfaqeeh et al., 2013; Vesela et al., 2019). From ED15, the bony crypt around the dental primordia starts to be remodeled, mostly by bone resorption (Radlanski et al., 2015).
The transition from the epithelial tooth bud to cap is accompanied by the differentiation of the former odontogenic mesenchymal condensation. This condensation gives rise to two compartments: (1) the dental papilla encapsulated by the epithelial cap, and (2) the dental follicle (or dental sac), which surrounds the epithelial cap and papilla (Figure 2). The papilla cells are progressively specified by sequential epithelial-mesenchymal interactions, and the preodontoblasts/odontoblasts lineage will later be controlled by the inner dental epithelium (Mina and Kollar, 1987; Lumsden, 1988; Ruch et al., 1995). The cell heterogeneity in the dental papilla becomes more and more complex (Keller et al., 2012; Kokten et al., 2014b; Krivanek et al., 2017). The characteristic shape and pattern of cells within the dental follicle allows its delineation from the surrounding loose interstitial mesenchyme (Figures 1H, 2).
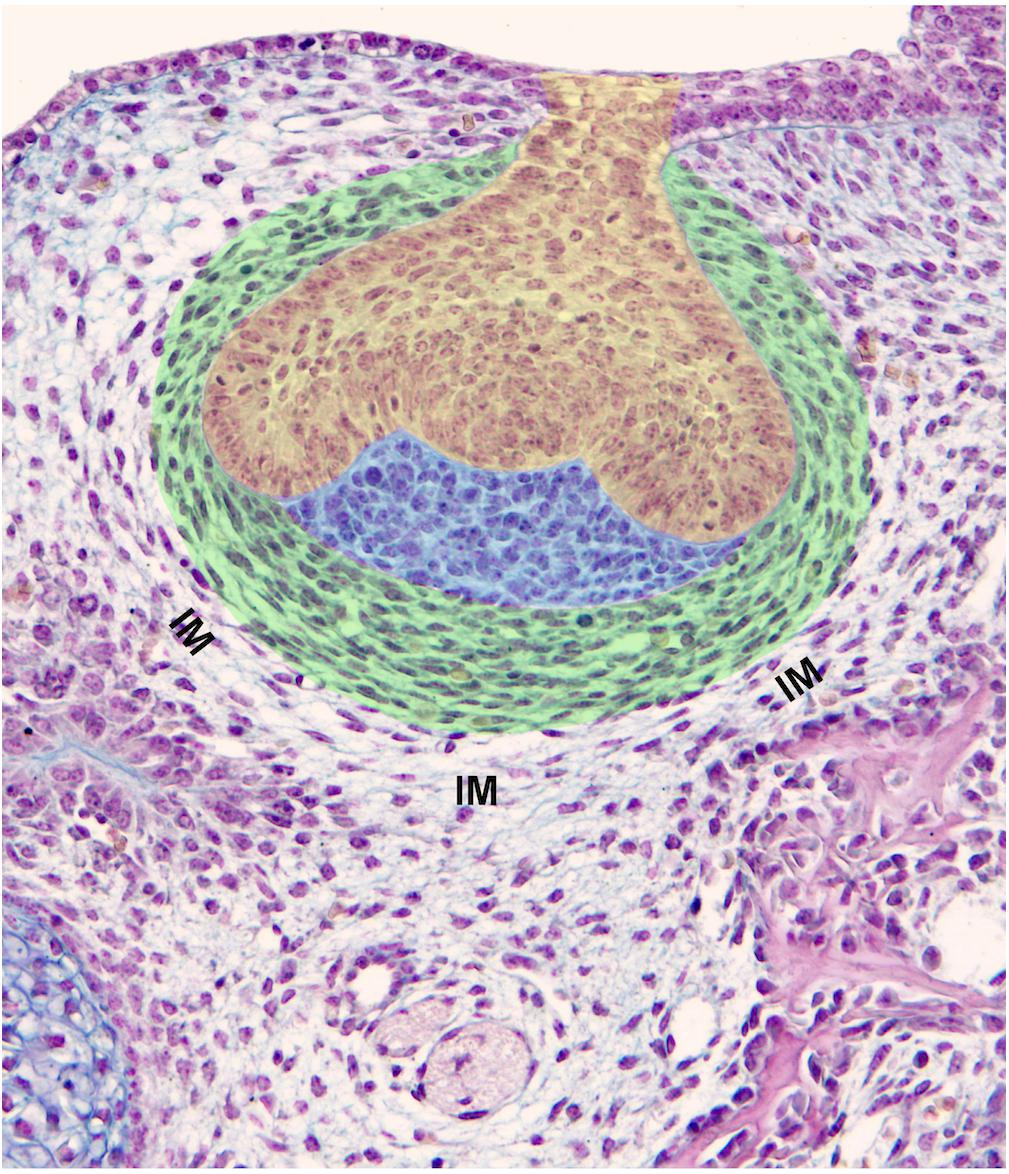
Figure 2. Components of a tooth germ and surrounding tissues. Mouse first lower molar germ at ED14.5 exhibits a clear subdivision of dental mesenchyme including the dental papilla (blue) and dental follicle/dental sac (green). Cells of the dental papilla show neither specific shape nor spatial organization. Conversely, cells of the dental sac show an elongated shape being arranged in concentric rings (green). The contemporaneous presence of increased cell density, their specific shape and pattern are criteria for the determination of the dental sac boundary. The dental epithelium is at a cap stage (yellow). IM, interstitial mesenchyme.
At ED14.5, dispersed cells of the interstitial mesenchyme are interposed between the external cells of the dental follicle and the osteogenic condensation (Figures 1G–I, 2). Very quickly, this mesenchyme shows further peculiarity. Immunostainings for VEGFR2, CD31, CD34 showed that the interstitial mesenchyme is vascularized at ED14, slightly before the dental mesenchyme and before the forming bone itself (Nait Lechguer et al., 2008; Keller et al., 2012; Vesela et al., 2019).
This interstitial mesenchyme is maintained at ED15, when the tooth germ has reached the late cap stage and bone has developed. How far these cells will later participate to the PDL and possibly even to the osteogenic mesenchyme is unknown since cell tracing has not been performed for this population (Zhao et al., 2013), in opposition to cranial neural crest cells (Chai et al., 2000), or mesenchymal cells facing the tip of the tooth bud (Rothova et al., 2012). Dissociation-reassociation experiments were performed using dissociated papilla and follicle cells from cap stage (ED14) molar germs (see Figure 1 in Kokten et al., 2014a). When re-associated with an intact enamel organ or dissociated epithelial cells, it was possible to reconstitute a whole tooth. This showed that although all initial positional information had been lost, mesenchymal cells at this stage can reconstitute a whole tooth germ. This demonstrated a high cell plasticity (Hu et al., 2005; Kuchler-Bopp et al., 2011), which decreases at later stages (Keller et al., 2011). This was in agreement with labeling and fate-mapping experiments showing that cells from the odontogenic condensation at the bud stage could participate in the follicle at the cap stage (Rothova et al., 2012). These experiments still raise the question of understanding why do these cells migrate, when cells of the follicle are already present there at that stage (Figure 2). One possibility is that the migrating dental mesenchymal cells increase the heterogeneity of the dental follicle and bring new necessary cell type(s) still absent before. It would thus be of interest to investigate in more details the heterogeneity of dental follicle cells before and after cell migration. Two other questions to be addressed concern: (1) the disruption of cell-cell/cell-matrix interactions within the dental papilla to allow cell migration and (2) the nature of the signals driving such a long distance migration and mediating cell targeting.
Cells from the interstitial mesenchyme (Figure 2), or at least a sub-population, are particularly important as possibly contributing to the development of the future PDL. At early developmental stage, and in opposition to the dental mesenchyme and follicle cells, interstitial mesenchymal cells do not express markers for NCDCs (Chai et al., 2000). It has been proposed that gingival connective tissue fibroblasts originate from perifollicular mesenchyme, a derivative of the stomodeal mesoderm (Cho and Garant, 2000). This particular origin of least part of the PDL fibroblasts might explain their specific properties and differences with the neighboring ecto-mesenchymal odontogenic and osteogenic condensations. Among these peculiarities, interstitial mesenchymal cells produce an extracellular matrix (ECM) that will not mineralize, thus impairing tooth ankylosis during later development.
Mechanisms Involved in Mesenchymal Cell Condensations
Cell condensation is a fundamental mechanism involved in morphogenesis. Condensations of mesenchymal cells take place during the development of almost all tissues and organs, including skin appendages, primordia of sensory organs, parenchymatous organs and musculo-skeletal structures (for review, see Giffin et al., 2019). Cell condensation occurs when a population of originally dispersed cells aggregates to differentiate into a specific cell/tissue type (Scheme 1; Hall and Miyake, 2000). The condensation results from altered mitotic activity, absence of centrifugal cell movement and/or centripetal cell aggregation (Hall and Miyake, 1995). According to Shyer et al. (2017), mesenchymal cells have an intrinsic potential to aggregate. The increase in cell density might facilitate cell–cell communication, possibly required for subsequent lineage acquisition (McKeown et al., 2005). However, the odontogenic and osteogenic mesenchymal condensations are separated by a non-condensed region. This illustrates early differences in the characteristics of mesenchymal cells, despite they are very next to each other. Each of these three cases reflect collective cell behavior, where mechanobiology might play an important role (Ladoux and Mège, 2017). The mesenchymal cell condensation, as it appears in early stage of odontogenesis and mandibular osteogenesis, precedes blood vessels ingrowth. This might be a general situation to be considered for regenerative medicine (Takebe et al., 2015). Furthermore, during tooth development, vascularization is anticipated in the interstitial mesenchyme, and blood vessels do not enter the condensed mesenchyme before the cap stage tooth germ as stated above. Similarly, blood vessels do not enter the osteogenic condensation before ED14 (Vesela et al., 2019).
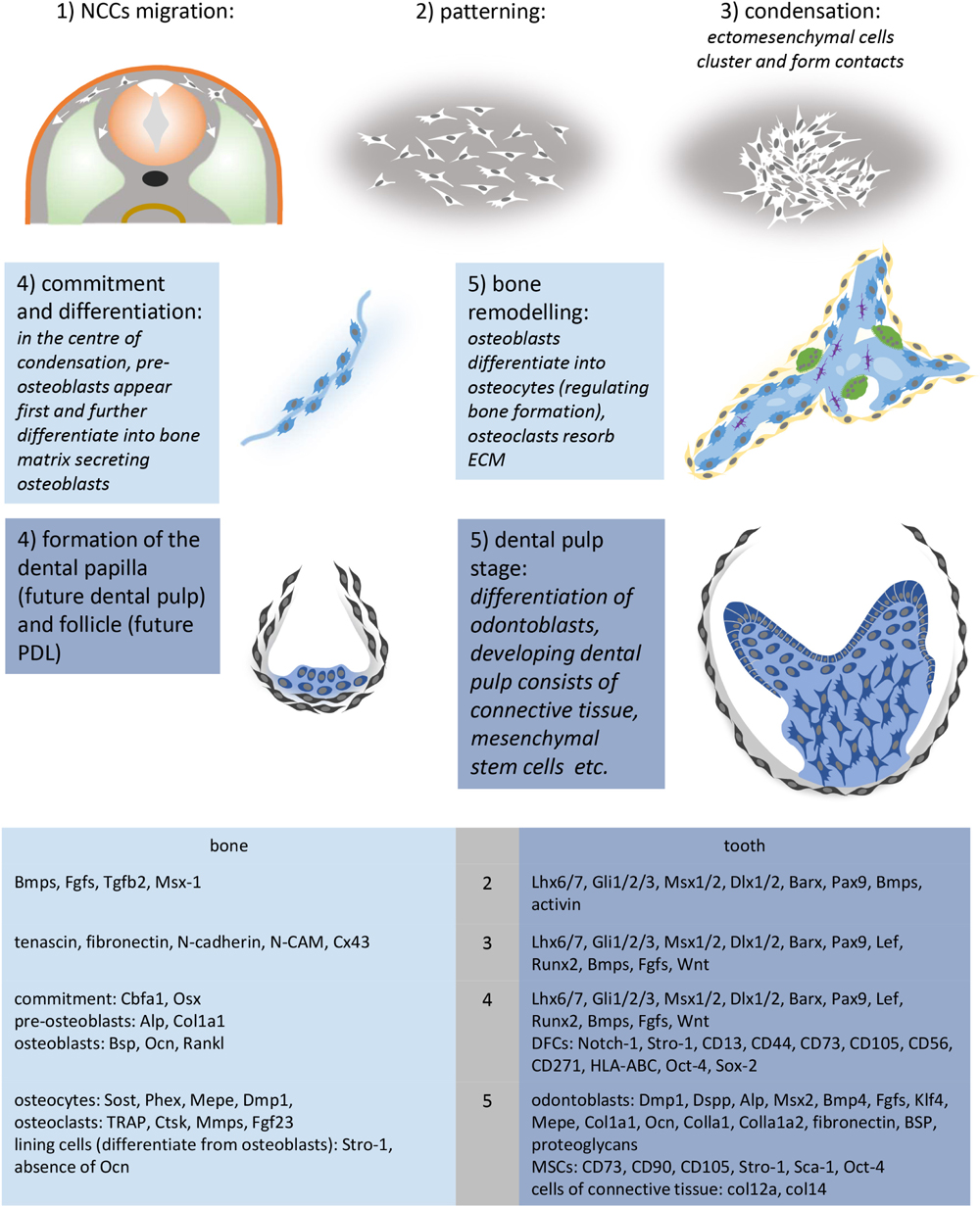
SCHEME 1. Crucial steps and molecules involved in the development of mesenchymal condensations. Molecular markers were summarized according to Casagrande et al. (2010), Dallas and Bonewald (2010), Everts et al. (2002), Ferguson and Atit (2019), Franz-Odendaal (2011), Giffin et al. (2019), Hall and Miyake (2000), Ledesma-Martínez et al. (2016), Lin et al., 2011, 2013; Nanci et al. (2008), Zhou et al. (2019), and Zvackova et al. (2017). Ectoderm (orange), NCCs (white), somite (light green), osteoblasts (light blue), lining cells – osteogenic cells (yellow), osteocytes (purple), osteoclasts (green), dental follicle (gray), dental papilla (dark blue), odontoblasts (dark blue-columnar).
Odontogenic Condensation
Condensation of the dental mesenchyme occurs during early steps of odontogenesis. Changes in the cell shape or size were suggested to possibly mediate this phenomenon (Mammoto et al., 2011, 2013). At this stage, histology did not confirm it, but showed change in cell density (Figures 1B,E). A decrease in intercellular spaces was obvious, which could be related to a change in either the ECM itself, in cell–cell interactions, or both. At the bud stage, syndecan and tenascin were detected within the odontogenic mesenchymal condensation and might be involved the in condensation process (Vainio and Thesleff, 1992; Thesleff et al., 1996). Collagen type VI, also present there, has been suggested to play a key role by stabilizing odontogenic cell condensation (Mammoto et al., 2015). According to these authors, cross-linking of collagen type VI by lysyl-oxidase would be involved and interfering with it would change the size of the condensation.
The condensation of the odontogenic mesenchyme appears to be regulated by signaling from the bud epithelium (Dassule and McMahon, 1998). Little is known about this signaling. Mammoto et al. (2011) showed that during mouse tooth development, FGF8 and (semaphorin) SEMA3F, produced by early dental epithelium, were causally involved in mesenchymal cell condensation. This mechanical compaction induced changes in the expression of transcription factors (PAX9, MSX1) and a growth factor (BMP4). These authors concluded that mechanical compression of the mesenchyme is necessary to induce tooth-specific cell fate although a condensation also takes place before osteogenic markers are expressed. The fact that one is in contact with an epithelium, while the other is not, is probably much more important. This has been very precisely documented in the developing tooth at later stage. In case of odontoblasts layer versus sub-odontoblastic cells, this is determined after the last cell division. Among the two daughter cells, the one in contact with a stage-specific basement membrane will differentiate as an odontoblast and elongate, while the other one will gets a flatten shape (Ruch et al., 1982, 1995; Ruch, 1998). However, in vitro experiments showed that growth factors, such as TGFbeta-1 or -3, when immobilized by heparin, can replace the inner dental epithelium and basement membrane, and induce preodontoblasts to odontoblasts differentiation (Ruch et al., 1995). BMP-2, -4, and -7 can induce it as well, although in more restricted areas. This involves a change in cell shape, elongation, as well as cytological and functional differentiation (polarized secretion of predentin), as it occurs in physiological conditions (Ruch, 1998).
In case of skin and follicle patterning in avian, Shyer et al. (2017) suggested an inverse mechanism, where mesenchymal cell condensation would change the organization of adjacent epidermal cells by triggering a mechanosensitive activation of beta-catenin in these cells.
Osteogenic Condensation
During development, a pre-osteogenic phase characterized by mesenchymal cell condensation (for review, see Hall and Miyake, 2000; Jabalee et al., 2013) is followed by post-condensation stage, including osteogenic cell differentiation, vascularization, innervation and mineralization of the ECM. However, these pre- and post-condensation stages remain connected (Dunlop and Hall, 1995). Although the mechanism mediating it remains unclear, mesenchymal cell condensation represents the initial stage of osteogenic specification. Hall and Miyake (2000) have proposed a series of molecules possibly involved in it (Scheme 1).
Fibroblast growth factors (FGFs) as well as bone morphogenetic proteins (BMPs) have been suggested to play a role in the initiation of condensation (Wu et al., 2007; Javed et al., 2010). Tissue cultures and recombinations in vitro showed that mandibular osteogenesis involves post-migratory neural crest-derived ecto-mesenchymal cells and their interaction with a mandibular epithelium (Hall, 1980; MacDonald and Hall, 2001). These experimental results raise interesting questions about the signaling process, given the long distance between the initially condensing osteogenic mesenchyme and epithelial tissues in situ.
The process of condensation itself requires changes in the local tissue mechanical properties (Mammoto et al., 2011). The generation of contractile forces within mesenchymal cells has been suspected (Oster et al., 1983; Ray and Chapman, 2015). Most of the data to support it and their role in mechanotransduction during osteogenesis have been obtained from in vitro experiments (Discher et al., 2005; Komatsu et al., 2018; Giffin et al., 2019). Concerning cell–cell interactions, three connexins, involved in the formation of gap junctions have been investigated during initial mandibular osteogenesis in the chick embryo. Among these, connexin43 (Cx43) appeared to be associated with the condensation of mesenchyme and the earliest stages of osteogenesis (Minkoff et al., 1994). Cx43 gene deletion in the mouse also leads to cell autonomous osteoblast dysfunction and delayed mineralization (Lecanda et al., 2000). Cx43-containing gap junctions thus seem to play an important role in the intercellular communication between the interconnected bone cells network (osteoprogenitor cells, osteoblast, and osteocytes) (Moorer et al., 2017). Similarly, the possible role of cadherins, which mediate interactions with the cytoskeleton of adjacent cells, has been investigated in vitro (Griffin et al., 2017). Adhesion junctions are mechanosensing structures, which play diverse roles. Among these, they regulate the expression of transcription factors during osteogenesis (Guntur et al., 2012). It would thus be of interest to investigate the pattern of cadherins during early mandibular/alveolar bone development and to compare it with the situation in neighbor interstitial mesenchyme.
Mesenchymes Specification and Differentiation During Development
All three mesenchymes (odontogenous, osteogenous, and intermediary) progressively consist in heterogeneous cell types, different in each case and changing during development. In all three cases, besides the presence/absence of NCDCs, exogenous cells take part in cell heterogeneity. Most of these exogenous cells enter together during vascularization, and later during innervation (Scheme 2).
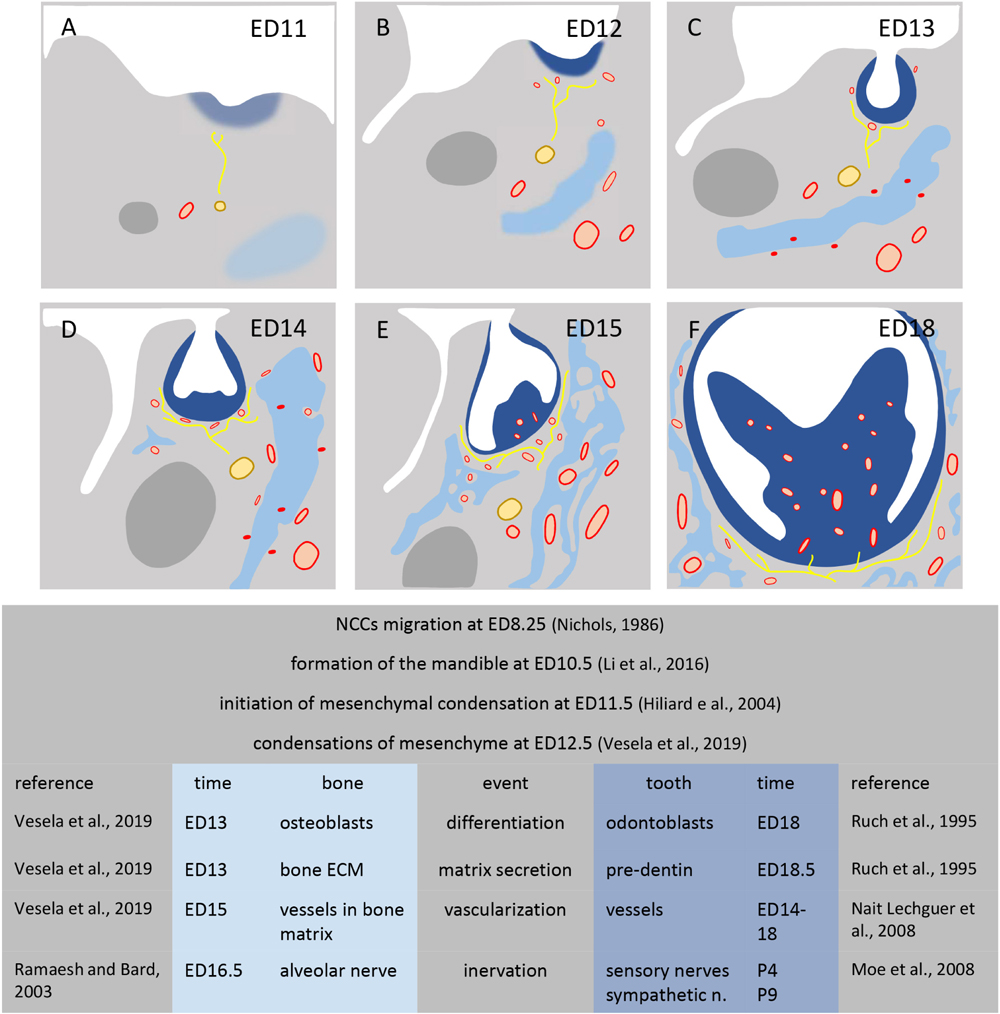
SCHEME 2. Development of mesenchymal condensations in mouse mandible. Figures show the mandible at stage ED11 (A), ED12 (B), ED13 (C), ED14 (D), ED15 (E), ED18 (F). Dental condensation (dark blue), bone condensation (light blue), Meckel’s cartilage (dark gray), nerves (yellow), vessels (red).
Dental Mesenchyme
The interactions of the odontogenic neural crest-derived mesenchymal cells with the oral epithelium determine a specific fate, very different from that of the other set of condensing osteogenic mesenchymal cells.
The dental papilla ecto-mesenchyme is clearly defined from the cap stage as part of condensed mesenchyme surrounded by the dental epithelium up to cervical loop, while the rest of the odontogenous mesenchyme gives rise to the dental follicle (Figure 2). Several successive steps lead to the histo-differentiation of the dental papilla and follicle mesenchymes, which are controlled by time- and space-specific epithelial-mesenchymal interactions (MacNeil and Thomas, 1993; Ruch et al., 1995; Thesleff et al., 1995; Miletich and Sharpe, 2003). The first odontoblasts differentiate at ED18. At post-natal day (PN) 1, they become positive for CD73, a 5′nucleotidase, which might be related to their functional differentiation. This 5′nucleotidase as well as alkaline phosphatase use AMP as a substrate (Andrade et al., 2008; Yegutkin, 2008) and could play a role in the expression of osteocalcin, which takes place in mature odontoblasts (Bidder et al., 1998). Odontoblasts were transiently positive for CD90 at PN1 and negative again at PN4 (Keller et al., 2012).
In opposition to clearly distinct cell compartments in the enamel organ, histology does not show anything similar in the forming dental papilla, except for preodontoblast/odontoblast, sub-odontoblastic layers and blood vessels.
Immunostaining revealed the cellular heterogeneity within the dental mesenchyme and implantation experiments in GFP mice were necessary to distinguish the endogenous/exogenous origin of these cells (Kuchler-Bopp et al., 2011; Keller et al., 2012; Kokten et al., 2014b). In the first lower molar, the timing of mesenchymal specification by the epithelium varies along the antero-posterior axis of the tooth and also along the buccal-lingual one (Nakatomi et al., 2013). In both cases, these local variations in the mesenchyme follow the pattern of cusps formation and, within one cusp, are controlled by the specific geometric determination in the inner dental epithelium (Obara and Lesot, 2007; Nakatomi et al., 2013).
Immunostaining for cell surface markers illustrated the cell heterogeneity with distinct patterns in the dental mesenchyme (Keller et al., 2012). The different cell populations also showed a differential timing in their appearance. Amongst these cells some are external to the dental papilla. At the cap stage, the first blood vessels penetrate the dental mesenchyme (Nait Lechguer et al., 2008) and their mesodermal origin has been reported (Rothova et al., 2011). When cultured tooth germs or dental cell–cells re-associations were implanted under the skin in GFP mice, both were re-vascularized. GFP-positive cells from the host were associated with blood vessels (i.e., cells expressing CD34, CD146, and later alpha-SMA) (Nait Lechguer et al., 2008). Complementary experiments showed that CD90-positive cells, and blood vessels associated Sca-1-positive cells can be either GFP-positive or negative, indicating that, in both cases, they still consist of heterogeneous populations, at least regarding their origins (Keller et al., 2012). The immunodetection of CD31, 34, and 146 showed that at ED14, while blood vessels were already present in the interstitial mesenchyme, they only started to enter the dental follicle. They were detected in the whole papilla mesenchyme at ED18 up to cells underlying the odontoblasts layer (Keller et al., 2012). Microvessels reaching the odontoblast layer at PN7 were detected after staining for CD34, collagen type IV or CD146 (Kokten et al., 2014b). Multiple double stainings showed that all three antigens were co-localized. These capillaries might be involved in the transport of calcium necessary for dentin mineralization (Bishop, 1987).
At ED18 also, CD45-positive cells were detected in the dental papilla and follicle, while CD90-positive cells were restricted to the papilla. Several dendritic cell types resident or not were present in the dental mesenchyme (Zhang et al., 2006; Keller et al., 2012). They included immunocompetent cells (Okiji et al., 1992, 1997; Kaneko et al., 2008), which can be recruited to participate in reparative processes (Jontell et al., 1998; Farges et al., 2003; Goldberg et al., 2008). From PN1, Sca-1 was detected in the dental pulp but still, very few cells were visualized. At PN4, this antigen was mainly expressed by cells associated to the largest blood vessels at the apical part of the papilla as also observed for alpha-SMA (Keller et al., 2012).
Sensory nerves enter the dental mesenchyme at PN3-4, while sympathetic nerves involved in vasoregulation penetrate this tissue much later, at PN9 (Tabata et al., 1998; Moe et al., 2008). Sensory and sympathetic axons can make contacts with dental pulp vessels, but most of them are sensory ones (Byers, 1984; Moe et al., 2008). In the odontoblast layer at PN7, axons were very frequently detected in close proximity with capillaries as seen after double staining for peripherin and either CD34 or collagen IV, or CD146 and confirmed by transmission electron microscopy (Kokten et al., 2014b). At PN7, when reached by axons, odontoblasts could function as mechanosensory cells (Allard et al., 2006; Magloire et al., 2009, 2010). Using confocal laser scanning microscopy, Farahani et al. (2012) showed that the heterogeneity of odontoblasts-associated cells increased precisely at that time.
Double staining for S100-beta and GFAP, two markers for glial cells, did not completely overlap, showing the existence of distinct glial cell types. S100-beta positive cells also showed more proximity with microvessels than GFAP-positive ones. Glial cells reached odontoblasts at PN7 (Kokten et al., 2014b). Schwann cells were present near the basal pole of odontoblasts from PN9, but not detected in the odontoblast layer before PN60 (Corpron and Avery, 1973).
Several different stem cell populations have been identified in the papilla and dental follicle, which also contribute to the cellular heterogeneity of these tissues (Shi et al., 2005; Yen and Sharpe, 2008; Yu et al., 2015; Sharpe, 2016). Genetic lineage tracing was performed to better understand the origin and properties of mesenchymal stem cells (MSCs) in vivo (Sharpe, 2016). When tested after transplantation, they were reported to exhibit different phenotypic and functional properties (for review, see Volponi and Sharpe, 2013).
Altogether, these results exemplify very dynamic changes in the heterogeneity of dental mesenchymal cells during odontogenesis. This illustrates a further progressive specification of papilla cells and the contribution of exogenous cells entering this mesenchyme from about ED14 (Moe et al., 2008; Keller et al., 2012; Krivanek et al., 2017), after it became vascularized (Chai et al., 2000; Nait Lechguer et al., 2008; Rothova et al., 2011; Feng et al., 2011).
Mandibular Bone Specification
Amongst bone cells, osteocalcin-positive osteoblasts are detected in the osteogenic condensation already at ED13 in mouse embryos. Osteoblasts directly differentiate from condensed ecto-mesenchymal cells and give rise to osteocytes. Parathormone (PTH) shares its membrane-bound parathyroid hormone 1 receptor (PTH1r) with parathyroid hormone-related protein (PTHrP). PTH1r and PTHrP are involved in osteoblast maturation in the mandibular/alveolar bone by stimulating osteocalcin expression (Martin, 2005; Bobek et al., 2020). At ED15, these osteocytes are PTH1r- and VDR-positive. Glucocorticoids are involved in the control of osteocyte differentiation (Alemi et al., 2018). Osteocytes, as forming a canalicular network, are thought to constitute a mechanosensory network, involved in mechanotransduction (Burger and Klein-Nulend, 1999; Bonewald and Johnson, 2008). LRP5, a co-receptor of Wnt, is essential for mechanotransduction, a mechanism by which cells convert mechanical energy into biochemical signals (Sawakami et al., 2006). Alveolar bone is a highly mechanoresponsive tissue. Primary cilia act as mechano- and chemosensors, transferring signals from the extracellular to intracellular compartments. Cilia also modulate the non-canonical Wnt signaling (Yavropoulou and Yovos, 2016).
Osteoclasts, responsible for bone resorption have precursors in the bone marrow. The migration of these precursors is thus associated to the vascularization. TRAP-positive mononucleated cells were detected when blood vessels start to penetrate the osteogenic condensation, at ED14 (Bobek et al., 2020). TRAP-positive osteoclasts are detected at ED14, and multinucleated osteoclasts at E15 (Bobek et al., 2020). Protocadherin-7 is involved in osteoclastogenesis by promoting cell-cell fusion (Nakamura et al., 2014). The activation of osteoclasts is mediated by RANKL produced by PTH-stimulated osteoblasts (Boyce and Xing, 2007). RANKL-RANK-OPG is an essential signaling network for osteoblast-osteoclast cross-talk (Nakagawa et al., 1998).
Besides bone cells stricto sensu, blood vessels penetrate the osteogenous condensation at ED14, and the bone structure is vascularized by ED15 (Vesela et al., 2019). As reported in the case of the dental papilla, blood vessels play a major role in bone homeostasis, in regulating the osteogenic microenvironment, bringing minerals, growth factors, and osteogenic progenitor cells (e.g., Grosso et al., 2017). Endothelial cells secrete osteogenic factors, such as BMP-2 and BMP-4, which support osteoblast differentiation. VEGF regulates in a dose dependent way the expression SEMA3A in endothelial cells. SEMA3A inhibits osteoclasts differentiation, thus stimulating bone development (Hayashi et al., 2012; Kenan et al., 2019). Conversely, the deletion of Vegf in Osterix-positive osteoblast precursors reduces mandibular ossification (Wang et al., 2007; Hill et al., 2015).
In human, the alveolar nerve issued from the trigeminal ganglion extends in the lower jaw before bone tissue forms and early stages of bone formation take place in close relationship with the mandibular nerve (Kjaer, 1990). Interactions between neurons and bone tissue have been documented in several models during skeletal development and repair. However, only scarce data are available about the innervation of the developing mandibular/alveolar bone. During development, CGRP (calcitonin gene-related peptide) is required for the formation of mandibular bone. When associated with blood vessels, it controls local blood flow (Hill and Elde, 1991). ISH showed that at ED14.5, Cgrp is expressed by mesenchymal cells surrounding the bone matrix and blood vessels (Maeda et al., 2017). Nerves, bone cells, and immune cells, influence bone homeostasis through a local secretion SEMA3A, which is involved in the regulation of osteoblastogenesis and osteoclastogenesis (Jabalee et al., 2013; Xu, 2014).
Schwann cells can play an active role in osteogenesis in the mandibular bone, but all studies were performed in case of distraction osteogenesis, a surgical separation of the jaw bone to elicit new bone growth in the gap (Farhadieh et al., 2003; Byun et al., 2008). It would thus be of interest to investigate the innervation during mandibular/alveolar bone formation in the mouse embryo, and compare what happens during distraction osteogenesis with what can be observed during development. A mouse model has been set up to investigate the nerve dependency of the mouse skeletal stem cell (mSSC), progenitor cells responsible for skeletal development and repair (Jones et al., 2019). According to these authors, the mandibular bone repair is compromised after inferior alveolar nerve disruption and denervation, as a result of functional deficiencies of mSSCs. A partial rescue of the denervated phenotype has been observed after Schwann cell transplantation and by Schwann cell-derived growth factors, suggesting that mSSCs would be dependent on paracrine factors secreted by Schwann cells (Jones et al., 2019).
Jaw bones contain very limited quantities of orofacial bone/bone-marrow-derived MSCs. Their isolation thus requires a specific methodology. When compared to long bone marrow-derived MSCs, orofacial bone/bone-marrow-derived MSCs demonstrated specific immunomodulatory properties and different capacities in vitro, thus appearing as a unique population of MSCs (Yamaza et al., 2011). In vitro experiments showed that Wnt/β-catenin signaling pathway is involved in the regulation of MSCs migration (He et al., 2018). In vitro experiments showed that the chemical and physical properties of the microenvironment modulate the commitment and fate of mesenchymal stromal/stem cells (MSSCs). Intermittent fluid shear stress, a potent and physiologically relevant mechanical stimulus, regulates osteogenic differentiation of MSCs through a transient receptor potential melastatin 7 (TRPM7). TRPM7 is a member of a family of TRP mechanosensitive cation channels with a cytosolic α-kinase domain that interferes with cytoskeletal rearrangements (Clark et al., 2006; Liu et al., 2015). TRP responds to intracellular and extracellular stimuli. Liu et al. (2015) suggested that TRPM7 has differential roles in endochondral and intramembranous ossification.
From the Dental Follicle to the Periodontal Ligament
Although the dental follicle and dental papilla have the same ecto-mesenchymal origin (Ten Cate, 1997), they soon diverge, showing distinct cell shape and arrangement (Figure 2). Cells initially located in the interstitial mesenchyme (Figure 2) participate in the formation of the PDL. Progenitor cells present in the dental follicle then give rise to cementoblasts, and possibly contribute to the alveolar bone osteoblasts (Diekwisch, 2002 and references therein; Diep et al., 2009; Dangaria et al., 2011).
Progressive Cell Heterogeneity in the Interstitial Mesenchyme
As stated above, cells from the odontogenic condensation at the bud stage later form the dental papilla in the epithelial cap. Some have been suggested to migrate and participate in the follicle at the cap and bell stages (Osborn and Price, 1988; Rothova et al., 2012). The interstitial mesenchyme is highly vascularized already at ED14 (Nait Lechguer et al., 2008; Keller et al., 2012). It also starts to be innervated at the cap stage (Scheme 2; Luukko and Kettunen, 2014). This very complex heterogeneous cell population is progressively set up from ED14. At the same time and later, several distinct stem cells have been described, which play complementary roles (Luan et al., 2006). SEMA3A has been detected in the dental follicle from ED15 (Wada et al., 2014). These authors suggested that, besides its important role in innervation (Moe et al., 2012; Luukko and Kettunen, 2014), SEMA3A might also induce the conversion of PDL cells into mesenchymal-stem-like cells (Wada et al., 2014; Tomokiyo et al., 2019).
Periodontal Ligament
The PDL forms and differentiates quite late postnatally. At PN4 (Figures 3A, A1, A2). Even at PN7 (Figures 3B, B1, B2), the developing PDL does not yet show any characteristic organization (Keller et al., 2015, Figure 28.3a).
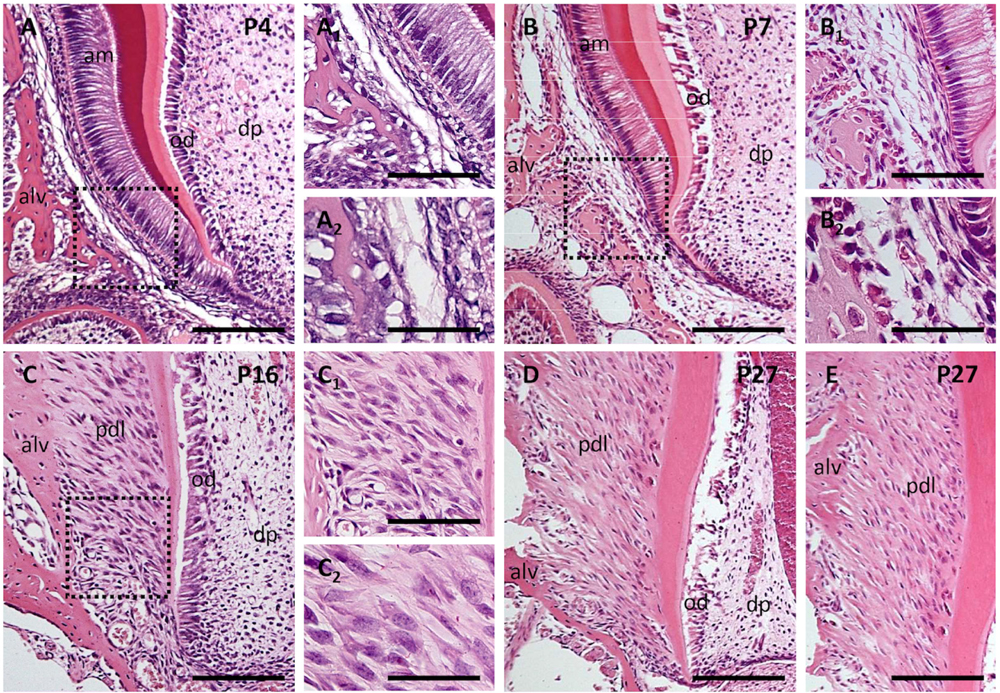
Figure 3. Postnatal development of periodontal ligaments at P4 (A,A1,A2), P7 (B,B1,B2), P16 (C,C1,C2), and P27 (D,E). Sections were stained by hematoxylin-eosin. Alv, alveolar bone; am, ameloblasts; dp, dental pulp; od, odontoblasts; pdl, periodontal ligament. The scale bar represents: 100 μm (in figures A–E); 50 μm (in figures A1,B1,C1); 25 μm (in figures A2,B2,C2).
The PDL consists of heterogeneous cell populations (e.g., Fleischmannova et al., 2010). These include fibroblasts (Lekic and McCulloch, 1996), vascular endothelial cells (Saghiri et al., 2018) and associated pericytes (Roguljic et al., 2013), macrophages (Epelman et al., 2014) and dendritic cells, acting as immunocompetent cells (Kaneko et al., 2008). Using the rat as a model, Kaneko et al. (2008) observed that the molecular phenotype of resident dendritic cells is heterogeneous, probably reflecting differences in their maturation/activation. From PN7 (Figures 3B, B1) to PN16 (Figures 3C, C1, C2) there is a striking increase in the density of PDL cells. A rapid growth of the PDL was reported to take place in between PN12 and PN16 (Lungova et al., 2011).
The ECM of the PDL consists in collagens, non-collagenous proteins and glycoproteins, enzymes such as MMPs and growth factors, such as FGFs, BMPs, platelet-derived growth factors (PDGFs). In vitro experiments showed that FGF2 inhibits the mineralization of the PDL matrix (Dangaria et al., 2009). The main role of the PDL is the anchoring of the tooth to the surrounding mandibular/alveolar bone and providing a space to allow tooth movement within the bone socket. This is mediated by bundles of collagen fibers ending as Sharpey’s fibers embedded in the cementum on one side and bone on the other (Figures 3D,E; Ho et al., 2007; Lee et al., 2015; Hirashima et al., 2020a). Based on immunoelectron microscopy, Huang et al. (1991) have proposed that periodontal and Sharpey’s fibers consist in co-fibrils of type I and type III collagens, which is supported by the similar flexibility of alfa-1 chains in both collagen types and their possible involvement in elastic energy storage during stretching (Silver et al., 2002). The PDL matrix also includes collagens types V, VI, and XII, as well as type XIV at late stage (Beertsen et al., 1997; Zvackova et al., 2017). Fibril-associated collagens with interrupted triple helices (FACITs) such as collagens XII and XIV are also involved in the regulation of collagen fibrillogenesis (Ricard-Blum, 2011). Transgenic mice carrying a mutation on collagen XII exhibit a disorganized arrangement of collagen fibers in the PDL (Reichenberger et al., 2000). Analyzing double KO mice, Wang et al. (2014) stressed the importance of small leucine-rich proteoglycans (SLRPs) in the maintenance of periodontal homeostasis, which involves the regulation of TGFβ/BMP signaling, matrix turnover, and collagen organization (Matheson et al., 2005; Wang et al., 2014; Zvackova et al., 2017).
The periodontal tissues are highly vascularized (Suphasiriroj et al., 2013; Lee et al., 2015) and close associations between blood vessels and sympathetic nerves allow their mediating vasoregulatory activities (Luukko and Kettunen, 2016). From PN4, immunostaining for peripherin showed the presence of axons in the PDL (Kokten et al., 2014a). Glial and Schwann cells are present in the PDL (Yamamoto et al., 2012; Kokten et al., 2014a). Phenotypic differences were also found when comparing Schwann cells in the pulp and PDL, based on their reactivity to antibodies to GFAP (Byers et al., 2004).
The PDL is exposed to various mechanical stresses associated with mastication. This leads to an active tissue remodeling, which involves progenitor cells (Hirashima et al., 2020a). Mechanical stimuli are important to orient the fate of stem cells. PDL stem cells (PDLSCs) can directly respond to mechanical forces. Both the ECM and mechanical forces can modulate the ability of PDLCs to sustain tissue development and maintenance (Nava et al., 2012; Sun et al., 2012). Heterogeneous populations of stem/progenitor cells have been identified there (Luan et al., 2006; Shinagawa-Ohama et al., 2017; Tomokiyo et al., 2018; Trubiani et al., 2019). PDLSCs showed osteogenic (Yang et al., 2017), cementogenic (Seo et al., 2004; Gauthier et al., 2017) and immunomodulatory properties (Wada et al., 2009). PDLSCs can also stimulate angiogenesis (Yeasmin et al., 2014; Amorim et al., 2016; Bae et al., 2017) or engage in neurogenesis (Ng et al., 2019). In case of angiogenesis, it seems a subset of PDLSCs (CD105+) may play a pivotal role, which would involve neuropilin-2 (NRP-2) (Amorim et al., 2016). PDLSCs can also contribute to the repair and regeneration of the periodontium (Tomokiyo et al., 2019).
Biological Interfaces: Spatial Limits for Mineralization
There are spatial limits for mineralizing areas in the tooth (predentin/dentin) as well as in the cementum/PDL and PDL/bone junctions (McKee and Nanci, 1996; Lausch et al., 2013; Foster et al., 2018). On the mesenchymal aspect of the tooth, the biological interface between predentin/dentin is controlled by the odontoblasts and this dynamic interface will be maintained all life long (Linde, 1995; Orsini et al., 2007).
Although very thin, the PDL is vascularized and innervated. Innervation is an important parameter to prevent tooth ankylosis (Fujiyama et al., 2004). As mentioned above, the PDL is a soft tissue exposed to important loadings when mediating tooth anchoring to the mandibular bone. The bone matrix, as well as the cementum, is mineralized while the PDL ECM is not. In both cases, this is controlled by specific matrix constituents (i.e., BSP, osteopontin versus PLAP1/asporin), being present or not (Steitz et al., 2002; Hakki et al., 2006; Yamada et al., 2007; Foster et al., 2018) and associated growth factors (Dangaria et al., 2009). Asporin is considered a negative regulator of mineralization (Leong et al., 2012). At their insertion sites into either the alveolar bone or cementum, the PDL fibers are mineralized (McKee et al., 2013). In vitro re-mineralization approaches have been designed to investigate the mechanism involved in it. These experiments showed that the ECM itself retains the necessary information to reproduce the spatial specificity of soft-mineralized tissues boundaries (Lausch et al., 2013).
These biological interfaces allow relative movements while resisting biomechanical loads (Ho et al., 2007). Their structural heterogeneity makes it difficult to investigate, and require the development of appropriated technologies together with adjusted methodologies (Boskey, 1998; Shahar and Weiner, 2018 and citations therein). For a simultaneous visualization of collagen fibrils, crystals, and intracellular components, Shahar and Weiner (2018) strongly favor 3D-imaging: complementary methods have been developed for that purpose. They include high resolution confocal light microscopy (Costello et al., 2016), high resolution Micro-CT (Burghardt et al., 2011), serial block face SEM (Denk and Horstmann, 2004), focused ion beam SEM (Hirashima et al., 2020b), each method having its own limitations. Although on a different model, backscattered electron (Kerschnitzki et al., 2011), second-harmonic generation imaging (Campagnola et al., 2002) and synchrotron small angle X-ray scattering (Groetsch et al., 2019) have been used to visualize collagen fibers crossing the soft/hard tissue junction (Paris et al., 2017). Besides structural aspects, experimental approaches allowed addressing questions about functional points of view, such as the relationship between mechanical strain amplification, mineral deposition/resorption and the dynamic correlation between these processes (Grandfield et al., 2015). In physiological conditions, mineral apposition is much more slow and regular. The transformation of amorphous to crystalline phase of mineral might allow rapid local changes, but has not yet been documented in detail.
Conclusion
Within the lower jaw of the mouse embryo, odontogenic and osteogenic condensations form almost simultaneously and next to each other. Although it has been published that mesenchymal cells have a natural tendency to aggregate, the two condensations remain separated by a loose interstitial mesenchyme. In opposition to the odontogenic and osteogenic ecto-mesenchymes originating from cranial NCDCs, cells from the interstitial mesenchyme do not express markers for neural crest origin. In all three zones, there is a progressive income of external cells: osteoclasts, cells mediating the vascularization or involved in the innervation, as well as stem/progenitor cells. Very soon all three mesenchymal areas show structural and physical differences. The secretion of distinct matrices will then support the different mineralization processes in odontogenic and osteogenic condensations. Besides its different origin, the interposed mesenchyme (prospective PDL) will not mineralize. This involves matrix-associated growth factors, some of which inhibit mineralization. These complementary processes lead to the formation of a functional complex: a tooth stabilized in a bone socket where movement still remains possible. While morphogenesis has been investigated in detail, mostly cellular aspects were taken into account in this review. Several questions remain, mainly concerning physical aspects within the three mesenchymes at early stages, such as the nature of differential cell-cell and cell-matrix interactions mediating cohesion as well as mechanosensing. Improving our knowledge about it would allow a better understanding of the mechanisms mediating/preventing the early mesenchymal cell segregation/compartmentalization and their condensation.
Author Contributions
HL and RP contributed to the conception and writing of the manuscript. RP, ES, and EM revised it critically. ES and RP performed the illustrations. ES, RP, EM, and HL approved the content of the manuscript for publication and agreed to be accountable for all aspects of the work. All authors contributed to the article and approved the submitted version.
Funding
This work was supported by the Czech Science Foundation (GACR project19-29667L) and by the Research Program Progress Q29 by Charles University.
Conflict of Interest
The authors declare that the research was conducted in the absence of any commercial or financial relationships that could be construed as a potential conflict of interest.
References
Alemi, A. S., Mazur, C. M., Fowler, T. M., Woo, J. J., Knott, P. D., and Alliston, T. (2018). Glucocorticoids cause mandibular bone fragility and suppress osteocyte perilacunar-canalicular remodeling. Bone Rep. 9, 145–153. doi: 10.1016/j.bonr.2018.09.004
Alfaqeeh, S. A., Gaete, M., and Tucker, A. S. (2013). Interactions of the tooth and bone during development. J. Dent. Res. 92, 1129–1135. doi: 10.1177/0022034513510321
Allard, B., Magloire, H., Couble, M. L., Maurin, J. C., and Bleicher, F. (2006). Voltage-gated sodium channels confer excitability to human odontoblasts: possible role in tooth pain transmission. J. Biol. Chem. 281, 29002–29010. doi: 10.1074/jbc.M601020200
Amorim, B. R., Silvério, K. G., Casati, M. Z., Sallum, E. A., Kantovitz, K. R., and Nociti, F. H. Jr. (2016). Neuropilin controls endothelial differentiation by mesenchymal stem cells from the periodontal ligament. J. Periodontol. 87, e138–e147. doi: 10.1902/jop.2016.150603
Andrade, C. M., Roesch, G. C., Wink, M. R., Guimarães, E. L., Souza, L. F., Jardim, F. R., et al. (2008). Activity and expression of ecto-5’-nucleotidase/CD73 are increased during phenotype conversion of a hepatic stellate cell line. Life Sci. 82, 21–29. doi: 10.1016/j.lfs.2007.10.003
Bae, Y. K., Kim, G. H., Lee, J. C., Seo, B. M., Joo, K. M., Lee, G., et al. (2017). The significance of SDF-1α-CXCR4 axis in in vivo angiogenic ability of human periodontal ligament stem cells. Mol. Cells 40, 386–392. doi: 10.14348/molcells.2017.0004
Beertsen, W., McCulloch, C. A., and Sodek, J. (1997). The periodontal ligament: a unique, multifunctional connective tissue. Periodontology 13, 20–40. doi: 10.1111/j.1600-0757.1997.tb00094.x
Berendsen, A. D., and Olsen, B. R. (2015). Bone development. Bone 80, 14–18. doi: 10.1016/j.bone.2015.04.035
Bidder, M., Latifi, T., and Towler, D. A. (1998). Reciprocal temporospatial patterns of Msx2 and Osteocalcin gene expression during murine odontogenesis. J. Bone. Miner. Res. 13, 609–619. doi: 10.1359/jbmr.1998.13.4.609
Bishop, M. A. (1987). An investigation of pulp capillaries and tight junctions between odontoblasts in cats. Anat. Embryol. 177, 131–138. doi: 10.1007/BF00572537
Bobek, J., Oralova, V., Lesot, H., Kratochvilova, A., Doubek, J., and Matalova, E. (2020). Onset of calciotropic receptors during the initiation of mandibular/alveolar bone formation. Ann. Anat. 227:151427. doi: 10.1016/j.aanat.2019.151427
Bonewald, L. F., and Johnson, M. L. (2008). Osteocytes, mechanosensing and Wnt signaling. Bone 42, 606–615. doi: 10.1016/j.bone.2007.12.224
Boskey, A. L. (1998). Biomineralization: conflicts, challenges, and opportunities. J. Cell Biochem. Suppl. 30-31, 83–91. doi: 10.1002/(sici)1097-4644(1998)72:30/31%2B<83::aid-jcb12>3.0.co;2-f
Boyce, B. F., and Xing, L. (2007). Biology of RANK, RANKL, and osteoprotegerin. Arthritis Res. Ther. 9(Suppl. 1), S1. doi: 10.1186/ar2165
Burger, E. H., and Klein-Nulend, J. (1999). Mechanotransduction in bone–role of the lacuno-canalicular network. FASEB J. 13(Suppl.) S101–S112.
Burghardt, A. J., Link, T. M., and Majumdar, S. (2011). High-resolution computed tomography for clinical imaging of bone microarchitecture. Clin. Orthop Relat. Res. 469, 2179–2193. doi: 10.1007/s11999-010-1766-x
Byers, M. R. (1984). Dental sensory receptors. Int. Rev. Neurobiol. 25, 39–94. doi: 10.1016/s0074-7742(08)60677-7
Byers, M. R., Maeda, T., Brown, A. M., and Westenbroek, R. E. (2004). GFAP immunoreactivity and transcription in trigeminal and dental tissues of rats and transgenic GFP/GFAP mice. Microsc. Res. Tech. 65, 295–307. doi: 10.1002/jemt.20130
Byun, J. H., Lee, J. H., Choi, Y. J., Kim, J. R., and Park, B. W. (2008). Co-expression of nerve growth factor and p75NGFR in the inferior alveolar nerve after mandibular distraction osteogenesis. Int. J. Oral Maxillofac. Surg. 37, 467–472. doi: 10.1016/j.ijom.2008.01.017
Campagnola, P. J., Millard, A. C., Terasaki, M., Hoppe, P. E., Malone, C. J., and Mohler, W. A. (2002). Three-dimensional high-resolution second-harmonic generation imaging of endogenous structural proteins in biological tissues. Biophys. J. 82, 493–508. doi: 10.1016/S0006-3495(02)75414-3
Casagrande, L., Demarco, F. F., Zhang, Z., Araujo, F. B., Shi, S., and Nör, J. E. (2010). Dentin-derived BMP-2 and odontoblast differentiation. J. Dent. Res. 89, 603–608. doi: 10.1177/0022034510364487
Chai, Y., Jiang, X., Ito, Y., Bringas, P. Jr., Han, J., Rowitch, D. H., et al. (2000). Fate of the mammalian cranial neural crest during tooth and mandibular morphogenesis. Development 127, 1671–1679.
Cho, M. I., and Garant, P. R. (2000). Development and general structure of the periodontium. Periodontol. 2000 24, 9–27. doi: 10.1034/j.1600-0757.2000.2240102.x
Clark, K., Langeslag, M., van Leeuwen, B., Ran, L., Ryazanov, A. G., Figdor, C. G., et al. (2006). TRPM7, a novel regulator of actomyosin contractility and cell adhesion. EMBO J. 25, 290–301. doi: 10.1038/sj.emboj.7600931
Corpron, R. E., and Avery, J. K. (1973). The ultrastructure of intradental nerves in developing mouse molars. Anat. Rec. 175, 585–606. doi: 10.1002/ar.1091750307
Costello, M. J., Brennan, L. A., Mohamed, A., Gilliland, K. O., Johnsen, S., and Kantorow, M. (2016). Identification and ultrastructural characterization of a novel nuclear degradation complex in differentiating lens fiber cells. PLoS One 11:e0160785. doi: 10.1371/journal.pone.0160785
Dallas, S. L., and Bonewald, L. F. (2010). Dynamics of the transition from osteoblast to osteocyte. Ann. N. Y. Acad. Sci. 1192, 437–443. doi: 10.1111/j.1749-6632.2009.05246.x
Dangaria, S. J., Ito, Y., Luan, X., and Diekwisch, T. G. (2011). Differentiation of neural-crest-derived intermediate pluripotent progenitors into committed periodontal populations involves unique molecular signature changes, cohort shifts, and epigenetic modifications. Stem Cells Dev. 20, 39–52. doi: 10.1089/scd.2010.0180
Dangaria, S. J., Ito, Y., Walker, C., Druzinsky, R., Luan, X., and Diekwisch, T. G. H. (2009). Extracellular matrix-mediated differentiation of periodontal progenitor cells. Differentiation 78, 79–90. doi: 10.1016/j.diff.2009.03.005
Dassule, H. R., and McMahon, A. P. (1998). Analysis of epithelial-mesenchymal interactions in the initial morphogenesis of the mammalian tooth. Dev. Biol. 202, 215–227. doi: 10.1006/dbio.1998.8992
Denk, W., and Horstmann, H. (2004). Serial block-face scanning electron microscopy to reconstruct three-dimensional tissue nanostructure. PLoS Biol. 2:e329. doi: 10.1371/journal.pbio.0020329
Diekwisch, T. G. (2002). Pathways and fate of migratory cells during late tooth organogenesis. Connect. Tissue Res. 43, 245–256.
Diep, L., Matalova, E., Mitsiadis, T. A., and Tucker, A. S. (2009). Contribution of the tooth bud mesenchyme to alveolar bone. J. Exp. Zool. B Mol. Dev. Evol. 312B, 510–517. doi: 10.1002/jez.b.21269
Discher, D. E., Janmey, P., and Wang, Y. L. (2005). Tissue cells feel and respond to the stiffness of their substrate. Science 310, 1139–1143. doi: 10.1126/science.1116995
Dunlop, L. L., and Hall, B. K. (1995). Relationships between cellular condensation, preosteoblast formation and epithelial-mesenchymal interactions in initiation of osteogenesis. Int. J. Dev. Biol. 39, 357–371.
Epelman, S., Lavine, K. J., and Randolph, G. J. (2014). Origin and functions of tissue macrophages. Immunity 41, 21–35. doi: 10.1016/j.immuni.2014.06.013
Everts, V., Delaisse, J. M., Korper, W., Jansen, D. C., Tigchelaar-Gutter, W., Saftig, P., et al. (2002). The bone lining cell: its role in cleaning Howship’s lacunae and initiating bone formation. J. Bone Miner. Res. 17, 77–90. doi: 10.1359/jbmr.2002.17.1.77
Farahani, R. M., Sarrafpour, B., Simonian, M., Li, Q., and Hunter, N. (2012). Directed glia-assisted angiogenesis in a mature neurosensory structure: pericytes mediate an adaptive response in human dental pulp that maintains blood-barrier function. J. Comp. Neurol. 520, 3803–3826. doi: 10.1002/cne.23162
Farges, J. C., Romeas, A., Melin, M., Pin, J. J., Lebecque, S., Lucchini, M., et al. (2003). TGF-beta1 induces accumulation of dendritic cells in the odontoblast layer. J. Dent. Res. 82, 652–656. doi: 10.1177/154405910308200816
Farhadieh, R. D., Nicklin, S., Yu, Y., Gianoutsos, M. P., and Walsh, W. R. (2003). The role of nerve growth factor and brain-derived neurotrophic factor in inferior alveolar nerve regeneration in distraction osteogenesis. J. Craniofac. Surg. 14, 859–865. doi: 10.1097/00001665-200311000-00007
Feng, J., Mantesso, A., De Bari, C., Nishiyama, A., and Sharpe, P. T. (2011). Dual origin of mesenchymal stem cells contributing to organ growth and repair. Proc. Natl. Acad. Sci. U.S.A. 108, 6503–6508. doi: 10.1073/pnas.1015449108
Ferguson, J. W., and Atit, R. P. (2019). A tale of two cities: the genetic mechanisms governing calvarial bone development. Genesis 57:e23248. doi: 10.1002/dvg.23248
Fleischmannova, J., Matalova, E., Sharpe, P. T., Misek, I., and Radlanski, R. J. (2010). Formation of the tooth-bone interface. J. Dent. Res. 89, 108–115. doi: 10.1177/0022034509355440
Foster, B. L., Ao, M., Salmon, C. R., Chavez, M. B., Kolli, T. N., Tran, A. B., et al. (2018). Osteopontin regulates dentin and alveolar bone development and mineralization. Bone 107, 196–207. doi: 10.1016/j.bone.2017.12.004
Franz-Odendaal, T. A. (2011). Induction and patterning of intramembranous bone. Front. Biosci. 16, 2734–2746. doi: 10.2741/3882
Fujiyama, K., Yamashiro, T., Fukunaga, T., Balam, T. A., Zheng, L., and Takano-Yamamoto, T. (2004). Denervation resulting in dento-alveolar ankylosis associated with decreased Malassez epithelium. J. Dent. Res. 83, 625–629. doi: 10.1177/154405910408300808
Gauthier, P., Yu, Z., Tran, Q. T., Bhatti, F. U., Zhu, X., and Huang, G. T. (2017). Cementogenic genes in human periodontal ligament stem cells are downregulated in response to osteogenic stimulation while upregulated by vitamin C treatment. Cell Tissue Res. 368, 79–92. doi: 10.1007/s00441-016-2513-8
Giffin, J. L., Gaitor, D., and Franz-Odendaal, T. A. (2019). The forgotten skeletogenic condensations: a comparison of early skeletal development amongst vertebrates. J. Dev. Biol. 7:4. doi: 10.3390/jdb7010004
Goldberg, M., Farges, J. C., Lacerda-Pinheiro, S., Six, N., Jegat, N., Decup, F., et al. (2008). Inflammatory and immunological aspects of dental pulp repair. Pharmacol. Res. 58, 137–147. doi: 10.1016/j.phrs.2008.05.013
Grandfield, K., Herber, R. P., Chen, L., Djomehri, S., Tam, C., Lee, J. H., et al. (2015). Strain-guided mineralization in the bone-PDL-cementum complex of a rat periodontium. Bone Rep. 3, 20–31. doi: 10.1016/j.bonr.2015.04.002
Griffin, F. E., Schiavi, J., McDevitt, T. C., McGarry, J. P., and McNamara, L. M. (2017). The role of adhesion junctions in the biomechanical behaviour and osteogenic differentiation of 3D mesenchymal stem cell spheroids. J. Biomech. 59, 71–79. doi: 10.1016/j.jbiomech.2017.05.014
Groetsch, A., Gourrier, A., Schwiedrzik, J., Sztucki, M., Beck, R. J., Shephard, J. D., et al. (2019). Compressive behaviour of uniaxially aligned individual mineralised collagen fibres at the micro- and nanoscale. Acta Biomater. 89, 313–329. doi: 10.1016/j.actbio.2019.02.053
Grosso, A., Burger, M. G., Lunger, A., Schaefer, D. J., Banfi, A., and Di Maggio, N. (2017). It takes two to tango: coupling of angiogenesis and osteogenesis for bone regeneration. Front. Bioeng. Biotechnol. 5:68. doi: 10.3389/fbioe.2017.00068
Guntur, A. R., Rosen, C. J., and Naski, M. C. (2012). N-cadherin adherens junctions mediate osteogenesis through PI3K signaling. Bone 50, 54–62. doi: 10.1016/j.bone.2011.09.036
Hakki, S. S., Wang, D., Franceschi, R. T., and Somerman, M. J. (2006). Bone sialoprotein gene transfer to periodontal ligament cells may not be sufficient to promote mineralization in vitro or in vivo. J. Periodontol. 77, 167–173. doi: 10.1902/jop.2006.050057
Hall, B. K. (1980). Tissue interactions and the initiation of osteogenesis and chondrogenesis in the neural crest-derived mandibular skeleton of the embryonic mouse as seen in isolated murine tissues and in recombinations of murine and avian tissues. J. Embryol. Exp. Morphol. 58, 251–264.
Hall, B. K., and Miyake, T. (1995). Divide, accumulate, differentiate: cell condensation skeletal development. Int. J. Dev. Biol. 39, 881–893.
Hall, B. K., and Miyake, T. (2000). All for one and one for all: condensations and the initiation of skeletal development. Bioessays 22, 138–147. doi: 10.1002/(sici)1521-1878(200002)22:2<138::aid-bies5>3.0.co;2-4
Hayashi, M., Nakashima, T., Taniguchi, M., Kodama, T., Kumanogoh, A., and Takayanagi, H. (2012). Osteoprotection by semaphorin 3A. Nature 485, 69–74. doi: 10.1038/nature11000
He, J., Zhang, N., Zhang, J., Jiang, B., and Wu, F. (2018). Migration critically meditates osteoblastic differentiation of bone mesenchymal stem cells through activating canonical Wnt signal pathway. Coll. Surf. B Biointerfaces 171, 205–213. doi: 10.1016/j.colsurfb.2018.07.017
Hill, C., Jacobs, B., Kennedy, L., Rohde, S., Zhou, B., Baldwin, S., et al. (2015). Cranial neural crest deletion of VEGFa causes cleft palate with aberrant vascular and bone development. Cell Tissue Res. 361, 711–722. doi: 10.1007/s00441-015-2150-7
Hill, E. L., and Elde, R. (1991). Distribution of CGRP-, VIP-, D beta H-, SP-, and NPY-immunoreactive nerves in the periosteum of the rat. Cell Tissue Res. 264, 469–480. doi: 10.1007/BF00319037
Hirashima, S., Kanazawa, T., Ohta, K., and Nakamura, K. I. (2020a). Three-dimensional ultrastructural analysis and histomorphometry of collagen bundles in the periodontal ligament using focused ion beam/scanning electron microscope tomography. J. Periodont. Res. 55, 23–23. doi: 10.1111/jre.12592
Hirashima, S., Kanazawa, T., Ohta, K., and Nakamura, K. I. (2020b). Three-dimensional ultrastructural imaging and quantitative analysis of the periodontal ligament. Anat. Sci. Int. 95, 1–11. doi: 10.1007/s12565-019-00502-5
Ho, S. P., Marshall, S. J., Ryder, M. I., and Marshall, G. W. (2007). The tooth attachment mechanism defined by structure, chemical composition and mechanical properties of collagen fibers in the periodontium. Biomaterials 28, 5238–5245. doi: 10.1016/j.biomaterials.2007.08.031
Hu, B., Nadiri, A., Bopp-Kuchler, S., Perrin-Schmitt, F., Wang, S., and Lesot, H. (2005). Dental epithelial histo-morphogenesis in the mouse: positional information versus cell history. Arch. Oral. Biol. 50, 131–136. doi: 10.1016/j.archoralbio.2004.09.007
Huang, W., Yang, S., Shao, J., and Li, Y. P. (2007). Signaling and transcriptional regulation in osteoblast commitment and differentiation. Front. Biosci. 12, 3068–3092. doi: 10.2741/2296
Huang, Y. H., Ohsaki, Y., and Kurisu, K. (1991). Distribution of type I and type III collagen in the developing periodontal ligament of mice. Matrix 11, 25–35. doi: 10.1016/s0934-8832(11)80224-6
Jabalee, J., Hillier, S., and Franz-Odendaal, T. A. (2013). An investigation of cellular dynamics during the development of intramembranous bones: the scleral ossicles. J. Anat. 223, 311–320. doi: 10.1111/joa.12095
Javed, A., Chen, H., and Ghori, F. Y. (2010). Genetic and transcriptional control of bone formation. Oral Maxillofac. Surg. Clin. North Am. 22, 283–293. doi: 10.1016/j.coms.2010.05.001
Jones, R. E., Salhotra, A., Robertson, K. S., Ransom, R. C., Foster, D. S., Shah, H. N., et al. (2019). Skeletal stem cell-schwann cell circuitry in mandibular repair. Cell Rep. 28, 2757–2766. doi: 10.1016/j.celrep.2019.08.021
Jontell, M., Okiji, T., Dahlgren, U., and Bergenholtz, G. (1998). Immune defense mechanisms of the dental pulp. Crit. Rev. Oral Biol. Med. 9, 179–200. doi: 10.1177/10454411980090020301
Kaneko, T., Okiji, T., Kaneko, R., and Suda, H. (2008). Characteristics of resident dendritic cells in various regions of rat periodontal ligament. Cell Tissue Res. 331, 413–421. doi: 10.1007/s00441-007-0539-7
Keller, L., Kökten, T., Kuchler-Bopp, S., and Lesot, H. (2015). “Tooth organ engineering,” in Stem Cell Biology and Tissue Engineering in Dental Sciences, eds A. Vishwakarma, P. Sharpe, S. Shi, X.-P. Wang, and M. Ramalingam (Cambridge, MA: Academic Press) 359–368. doi: 10.1016/B978-0-12-397157-9.00032-1
Keller, L. V., Kuchler-Bopp, S., and Lesot, H. (2012). Restoring physiological cell heterogeneity in the mesenchyme during tooth engineering. Int. J. Dev. Biol. 56, 737–746. doi: 10.1387/ijdb.120076hl
Keller, L. V., Kuchler-Bopp, S., Mendoza, S. A., Poliard, A., and Lesot, H. (2011). Tooth engineering: searching for dental mesenchymal cells sources. Front. Physiol. 2:7. doi: 10.3389/fphys.2011.00007
Kenan, S., Onur, ÖD., Solakoðlu, S., Kotil, T., Ramazanoðlu, M., Çelik, H. H., et al. (2019). Investigation of the effects of semaphorin 3A on new bone formation in a rat calvarial defect model. J. Craniomaxillofac. Surg. 47, 473–483. doi: 10.1016/j.jcms.2018.12.010
Kerschnitzki, M., Wagermaier, W., Roschger, P., Seto, J., Shahar, R., Duda, G. N., et al. (2011). The organization of the osteocyte network mirrors the extracellular matrix orientation in bone. J. Struct. Biol. 173, 303–311. doi: 10.1016/j.jsb.2010.11.014
Kjaer, I. (1990). Correlated appearance of ossification and nerve tissue in human fetal jaws. J. Craniofac. Genet. Dev. Biol. 10, 329–336.
Kokten, T., Bécavin, T., Keller, L., Weickert, J. L., Kuchler-Bopp, S., and Lesot, H. (2014a). Immunomodulation stimulates the innervation of engineered tooth organ. PLoS One 9:e86011. doi: 10.1371/journal.pone.0086011
Kokten, T., Lesot, H., and Kuchler-Bopp, S. (2014b). “Experimental design for the innervation of tooth forming from implanted cell re-associations,” in Cells and Biomaterials in Regenerative Medicine, ed. D. Eberli (Rijeka: InTech Open Access Publisher), 345–373.
Komatsu, N., Kajiya, M., Motoike, S., Takewaki, M., Horikoshi, S., Iwata, T., et al. (2018). Type I collagen deposition via osteoinduction ameliorates YAP/TAZ activity in 3D floating culture clumps of mesenchymal stem cell/extracellular matrix complexes. Stem Cell Res. Ther. 9:342. doi: 10.1186/s13287-018-1085-9
Krivanek, J., Adameyko, I., and Fried, K. (2017). Heterogeneity and developmental connections between cell types inhabiting teeth. Front. Physiol. 8:376. doi: 10.3389/fphys.2017.00376
Kuchler-Bopp, S., Keller, L., Poliard, A., and Lesot, H. (2011). “Tooth organ engineering: biological constraints specifying experimental approaches,” in Tissue Engineering for Tissue and Organ Regeneration, ed. D. Eberli (London: InTech), 317–346. doi: 10.5772/23483
Ladoux, B., and Mège, R. M. (2017). Mechanobiology of collective cell behaviours. Nat. Rev. Mol. Cell Biol. 18, 743–757. doi: 10.1038/nrm.2017.98
Lausch, A. J., Quan, B. D., Miklas, J. W., and Sone, E. D. (2013). Extracellular matrix control of collagen mineralization in vitro. Adv. Funct. Mater. 23, 4906–4912. doi: 10.1002/adfm.201203760
Lecanda, F., Warlow, P. M., Sheikh, S., Furlan, F., Steinberg, T. H., and Civitelli, R. (2000). Connexin43 deficiency causes delayed ossification, craniofacial abnormalities, and osteoblast dysfunction. J. Cell Biol. 151, 931–944. doi: 10.1083/jcb.151.4.931
Ledesma-Martínez, E., Mendoza-Núñez, V. M., and Santiago-Osorio, E. (2016). Mesenchymal stem cells derived from dental pulp: a review. Stem Cells Int. 2016:4709572. doi: 10.1155/2016/4709572
Lee, J. H., Pryce, B. A., Schweitzer, R., Ryder, M. I., and Ho, S. P. (2015). Differentiating zones at periodontal ligament-bone and periodontal ligament-cementum entheses. J. Periodontal Res. 50, 870–880. doi: 10.1111/jre.12281
Lekic, P., and McCulloch, C. A. (1996). Relationship of cellular proliferation to expression of osteopontin and bone sialoprotein in regenerating rat periodontium. Cell Tissue Res. 285, 491–500. doi: 10.1007/s004410050665
Leong, N. L., Hurng, J. M., Djomehri, S. I., Gansky, S. A., Ryder, M. I., and Ho, S. P. (2012). Age-related adaptation of bone-PDL-tooth complex: rattus-norvegicus as a model system. PLoS One 7:e35980. doi: 10.1371/journal.pone.0035980
Lin, H., Liu, H., Sun, Q., Yuan, G., Zhang, L., and Chen, Z. (2013). KLF4 promoted odontoblastic differentiation of mouse dental papilla cells via regulation of DMP1. J. Cell. Physiol. 228, 2076–2085. doi: 10.1002/jcp.24377
Lin, H., Xu, L., Liu, H., Sun, Q., Chen, Z., Yuan, G., et al. (2011). KLF4 promotes the odontoblastic differentiation of human dental pulp cells. J Endod. 37, 948–954. doi: 10.1016/j.joen.2011.03.030
Linde, A. (1995). Dentin mineralization and the role of odontoblasts in calcium transport. Connect. Tissue Res. 33, 163–170. doi: 10.3109/03008209509016997
Liu, Y. S., Liu, Y. A., Huang, C. J., Yen, M. H., Tseng, C. T., Chien, S., et al. (2015). Mechanosensitive TRPM7 mediates shear stress and modulates osteogenic differentiation of mesenchymal stromal cells through Osterix pathway. Sci. Rep. 5:16522. doi: 10.1038/srep16522
Luan, X., Ito, Y., Dangaria, S., and Diekwisch, T. G. (2006). Dental follicle progenitor cell heterogeneity in the developing mouse periodontium. Stem Cells Dev. 15, 595–608. doi: 10.1089/scd.2006.15.595
Lumsden, A. G. (1988). Spatial organization of the epithelium and the role of neural crest cells in the initiation of the mammalian tooth germ. Development 103(Suppl.) 155–169.
Lungova, V., Radlanski, R. J., Tucker, A. S., Renz, H., Misek, I., and Matalova, E. (2011). Tooth-bone morphogenesis during postnatal stages of mouse first molar development. J. Anat. 218, 699–716. doi: 10.1111/j.1469-7580.2011.01367.x
Luukko, K., and Kettunen, P. (2014). Coordination of tooth morphogenesis and neuronal development through tissue interactions: lessons from mouse models. Exp. Cell Res. 325, 72–77. doi: 10.1016/j.yexcr.2014.02.029
Luukko, K., and Kettunen, P. (2016). Integration of tooth morphogenesis and innervation by local tissue interactions, signaling networks, and semaphorin 3A. Cell Adh. Migr. 10, 618–626. doi: 10.1080/19336918.2016.1216746
MacDonald, M. E., and Hall, B. K. (2001). Altered timing of the extracellular-matrix-mediated epithelial-mesenchymal interaction that initiates mandibular skeletogenesis in three inbred strains of mice: development, heterochrony, and evolutionary change in morphology. J. Exp. Zool. 291, 258–273. doi: 10.1002/jez.1102.abs
MacNeil, R. L., and Thomas, H. F. (1993). Development of the murine periodontium. II. Role of the epithelial root sheath in formation of the periodontal attachment. J. Periodontol. 64, 285–291. doi: 10.1902/jop.1993.64.4.285
Maeda, Y., Miwa, Y., and Sato, I. (2017). Expression of CGRP, vasculogenesis and osteogenesis associated mRNAs in the developing mouse mandible and tibia. Ann. Anat. 221, 38–47. doi: 10.4081/ejh.2017.2750
Magloire, H., Couble, M. L., Thivichon-Prince, B., Maurin, J. C., and Bleicher, F. (2009). Odontoblast: a mechano-sensory cell. J. Exp. Zool. B Mol. Dev. Evol. 312B, 416–424. doi: 10.1002/jez.b.21264
Magloire, H., Maurin, J. C., Couble, M. L., Shibukawa, Y., Tsumura, M., Thivichon-Prince, B., et al. (2010). Topical review. Dental pain and odontoblasts: facts and hypotheses. J. Orofac. Pain 24, 335–349.
Mammoto, T., Mammoto, A., and Ingber, D. E. (2013). Mechanobiology and developmental control. Annu. Rev. Cell Dev. Biol. 29, 27–61. doi: 10.1146/annurev-cellbio-101512-122340
Mammoto, T., Mammoto, A., Jiang, A., Jiang, E., Hashmi, B., and Ingber, D. E. (2015). Mesenchymal condensation-dependent accumulation of collagen VI stabilizes organ-specific cell fates during embryonic tooth formation. Dev. Dyn. 244, 713–723. doi: 10.1002/dvdy.24264
Mammoto, T., Mammoto, A., Torisawa, Y. S., Tat, T., Gibbs, A., Derda, R., et al. (2011). Mechanochemical control of mesenchymal condensation and embryonic tooth organ formation. Dev. Cell 21, 758–769. doi: 10.1016/j.devcel.2011.07.006
Martin, T. J. (2005). Osteoblast-derived PTHrP is a physiological regulator of bone formation. J. Clin. Invest. 115, 2322–2324. doi: 10.1172/JCI26239
Matheson, S., Larjava, H., and Häkkinen, L. (2005). Distinctive localization and function for lumican, fibromodulin and decorin to regulate collagen fibril organization in periodontal tissues. J. Periodontal Res. 40, 312–324. doi: 10.1111/j.1600-0765.2005.00800.x
McKee, M. D., Hoac, B., Addison, W. N., Barros, N. M., Millán, J. L., and Chaussain, C. (2013). Extracellular matrix mineralization in periodontal tissues: noncollagenous matrix proteins, enzymes, and relationship to hypophosphatasia and X-linked hypophosphatemia. Periodontol. 2000 63, 102–122. doi: 10.1111/prd.12029
McKee, M. D., and Nanci, A. (1996). Osteopontin at mineralized tissue interfaces in bone, teeth, and osseointegrated implants: ultrastructural distribution and implications for mineralized tissue formation, turnover, and repair. Microsc. Res. Tech. 3, 141–164. doi: 10.1002/(sici)1097-0029(19960201)33:2<141::aid-jemt5>3.0.co;2-w
McKeown, S. J., Newgreen, D. F., and Farlie, P. G. (2005). Dlx2 over-expression regulates cell adhesion and mesenchymal condensation in ectomesenchyme. Dev. Biol. 281, 22–37. doi: 10.1016/j.ydbio.2005.02.004
Miletich, I., and Sharpe, P. T. (2003). Normal and abnormal dental development. Hum. Mol. Genet. 12, R69–R73. doi: 10.1093/hmg/ddg085
Mina, M., and Kollar, E. J. (1987). The induction of odontogenesis in non-dental mesenchyme combined with early murine mandibular arch epithelium. Arch. Oral. Biol. 32, 123–127. doi: 10.1016/0003-9969(87)90055-0
Minkoff, R., Rundus, V. R., Parker, S. B., Hertzberg, E. L., Laing, J. G., and Beyer, E. C. (1994). Gap junction proteins exhibit early and specific expression during intramembranous bone formation in the developing chick mandible. Anat. Embryol. 190, 231–241. doi: 10.1007/BF00234301
Moe, K., Kettunen, P., Kvinnsland, I. H., and Luukko, K. (2008). Development of the pioneer sympathetic innervation into the dental pulp of the mouse mandibular first molar. Arch. Oral. Biol. 53, 865–873. doi: 10.1016/j.archoralbio.2008.03.004
Moe, K., Sijaona, A., Shrestha, A., Kettunen, P., Taniguchi, M., and Luukko, K. (2012). Semaphorin 3A controls timing and patterning of the dental pulp innervation. Differentiation 84, 371–379. doi: 10.1016/j.diff.2012.09.003
Moorer, M. C., Hebert, C., Tomlinson, R. E., Iyer, S. R., Chason, M., and Stains, J. P. (2017). Defective signaling, osteoblastogenesis and bone remodeling in a mouse model of connexin 43 C-terminal truncation. J. Cell Sci. 130, 531–540. doi: 10.1242/jcs.197285
Nait Lechguer, A., Kuchler-Bopp, S., Hu, B., Haïkel, Y., and Lesot, H. (2008). Vascularization of engineered teeth. J. Dent. Res. 87, 1138–1143. doi: 10.1177/154405910808701216
Nakagawa, N., Kinosaki, M., Yamaguchi, K., Shima, N., Yasuda, H., Yano, K., et al. (1998). RANK is the essential signaling receptor for osteoclast differentiation factor in osteoclastogenesis. Biochem. Biophys. Res. Commun. 253, 395–400. doi: 10.1006/bbrc.1998.9788
Nakamura, H., Nakashima, T., Hayashi, M., Izawa, N., Yasui, T., Aburatani, H., et al. (2014). Global epigenomic analysis indicates protocadherin-7 activates osteoclastogenesis by promoting cell-cell fusion. Biochem. Biophys. Res. Commun. 455, 305–311. doi: 10.1016/j.bbrc.2014.11.009
Nakatomi, M., Hovorakova, M., Gritli-Linde, A., Blair, H. J., MacArthur, K., Peterka, M., et al. (2013). Evc regulates a symmetrical response to Shh signaling in molar development. J. Dent. Res. 92, 222–228. doi: 10.1177/0022034512471826
Nanci, A., Wazen, R., Nishio, C., and Zalzal, S. F. (2008). Immunocytochemistry of matrix proteins in calcified tissues: functional biochemistry on section. Eur. J. Histochem. 52, 201–214. doi: 10.4081/1218
Nava, M. M., Raimondi, M. T., and Pietrabissa, R. (2012). Controlling self-renewal and differentiation of stem cells via mechanical cues. J. Biomed. Biotechnol. 2012:797410. doi: 10.1155/2012/797410
Ng, T. K., Yang, Q., Fortino, V. R., Lai, N. Y., Carballosa, C. M., Greenberg, J. M., et al. (2019). MicroRNA-132 directs human periodontal ligament-derived neural crest stem cell neural differentiation. J. Tissue Eng. Regen. Med. 13, 12–24. doi: 10.1002/term.2759
Obara, N., and Lesot, H. (2007). Asymmetrical growth, differential cell proliferation, and dynamic cell rearrangement underlie epithelial morphogenesis in mouse molar development. Cell Tissue Res. 330, 461–473. doi: 10.1007/s00441-007-0502-7
Okiji, T., Jontell, M., Belichenko, P., Bergenholtz, G., and Dahlström, A. (1997). Perivascular dendritic cells of the human dental pulp. Acta Physiol. Scand. 159, 163–169. doi: 10.1046/j.1365-201X.1997.584337000.x
Okiji, T., Kawashima, N., Kosaka, T., Matsumoto, A., Kobayashi, C., and Suda, H. (1992). An immunohistochemical study of the distribution of immunocompetent cells, especially macrophages and Ia antigen-expressing cells of heterogeneous populations, in normal rat molar pulp. J. Dent. Res 71, 1196–1202. doi: 10.1177/00220345920710051201
Orsini, G., Ruggeri, A. Jr., Mazzoni, A., Papa, V., Mazzotti, G., Di Lenarda, R., et al. (2007). Immunohistochemical identification of decorin and biglycan in human dentin: a correlative field emission scanning electron microscopy/transmission electron microscopy study. Calcif. Tissue Int. 81, 39–45. doi: 10.1007/s00223-007-9027-z
Osborn, J. W., and Price, D. G. (1988). An autoradiographic study of periodontal development in the mouse. J. Dent. Res. 67, 455–461. doi: 10.1177/00220345880670020401
Oster, G. F., Murray, J. D., and Harris, A. K. (1983). Mechanical aspects of mesenchymal morphogenesis. J. Embryol. Exp. Morphol. 78, 83–125.
Paris, M., Götz, A., Hettrich, I., Bidan, C. M., Dunlop, J. W. C., Razi, H., et al. (2017). Scaffold curvature-mediated novel biomineralization process originates a continuous soft tissue-to-bone interface. Acta Biomater. 60, 64–80. doi: 10.1016/j.actbio.2017.07.029
Radlanski, R. J., Renz, H., Zimmermann, C. A., Mey, R., and Matalova, E. (2015). Morphogenesis of the compartmentalizing bone around the molar primordia in the mouse mandible during dental developmental stages between lamina, bell-stage, and root formation (E13-P20). Ann. Anat. 200, 1–14. doi: 10.1016/j.aanat.2015.01.003
Ray, P., and Chapman, S. C. (2015). Cytoskeletal reorganization drives mesenchymal condensation and regulates downstream molecular signaling. PLoS One 10:e0134702. doi: 10.1371/journal.pone.0134702
Reichenberger, E., Baur, S., Sukotjo, C., Olsen, B. R., Karimbux, N. Y., and Nishimura, I. (2000). Collagen XII mutation disrupts matrix structure of periodontal ligament and skin. J. Dent. Res. 79, 1962–1968. doi: 10.1177/00220345000790120701
Ricard-Blum, S. (2011). The collagen family. Cold Spring Harb. Perspect. Biol. 3:a004978. doi: 10.1101/cshperspect.a004978
Roguljic, H., Matthews, B. G., Yang, W., Cvija, H., Mina, M., and Kalajzic, I. (2013). In vivo identification of periodontal progenitor cells. J. Dent. Res. 92, 709–715. doi: 10.1177/0022034513493434
Rothova, M., Feng, J., Sharpe, P. T., Peterkova, R., and Tucker, A. S. (2011). Contribution of mesoderm to the developing dental papilla. Int. J. Dev. Biol. 55, 59–64. doi: 10.1387/ijdb.103083mr
Rothova, M., Peterkova, R., and Tucker, A. S. (2012). Fate map of the dental mesenchyme: dynamic development of the dental papilla and follicle. Dev. Biol. 366, 244–254. doi: 10.1016/j.ydbio.2012.03.018
Ruch, J. V. (1998). Odontoblast commitment and differentiation. Biochem. Cell Biol. 76, 923–938. doi: 10.1139/o99-008
Ruch, J. V., Lesot, H., and Bègue-Kirn, C. (1995). Odontoblast differentiation. Int. J. Dev. Biol. 39, 51–68.
Ruch, J. V., Lesot, H., Karcher-Djuricic, V., Meyer, J. M., and Olive, M. (1982). Facts and hypotheses concerning the control of odontoblast differentiation. Differentiation 21, 7–12. doi: 10.1111/j.1432-0436.1982.tb01187.x
Saghiri, M. A., Asatourian, A., Sorenson, C. M., and Sheibani, N. (2018). Mice dental pulp and periodontal ligament endothelial cells exhibit different proangiogenic properties. Tissue Cell. 50, 31–36. doi: 10.1016/j.tice.2017.11.004
Santagati, F., and Rijli, F. M. (2003). Cranial neural crest and the building of the vertebrate head. Nat. Rev. Neurosci. 4, 806–818. doi: 10.1038/nrn1221
Sawakami, K., Robling, A. G., Ai, M., Pitner, N. D., Liu, D., Warden, S. J., et al. (2006). The Wnt co-receptor LRP5 is essential for skeletal mechanotransduction but not for the anabolic bone response to parathyroid hormone treatment. J. Biol. Chem. 281, 23698–23711. doi: 10.1074/jbc.M601000200
Seo, B. M., Miura, M., Gronthos, S., Bartold, P. M., Batouli, S., Brahim, J., et al. (2004). Investigation of multipotent postnatal stem cells from human periodontal ligament. Lancet 364, 149–155. doi: 10.1016/S0140-6736(04)16627-0
Shahar, R., and Weiner, S. (2018). Open questions on the 3D structures of collagen containing vertebrate mineralized tissues: a perspective. J. Struct. Biol. 201, 187–198. doi: 10.1016/j.jsb.2017.11.008
Sharpe, P. T. (2016). Dental mesenchymal stem cells. Development 143, 2273–2280. doi: 10.1242/dev.134189
Shi, S., Bartold, P. M., Miura, M., Seo, B. M., Robey, P. G., and Gronthos, S. (2005). The efficacy of mesenchymal stem cells to regenerate and repair dental structures. Orthod. Craniofac. Res. 8, 191–199. doi: 10.1111/j.1601-6343.2005.00331.x
Shinagawa-Ohama, R., Mochizuki, M., Tamaki, Y., Suda, N., and Nakahara, T. (2017). Heterogeneous human periodontal ligament-committed progenitor and stem cell populations exhibit a unique cementogenic property under in vitro and in vivo conditions. Stem Cells Dev. 26, 632–645. doi: 10.1089/scd.2016.0330
Shyer, A. E., Rodrigues, A. R., Schroeder, G. G., Kassianidou, E., Kumar, S., and Harland, R. M. (2017). Emergent cellular self-organization and mechanosensation initiate follicle pattern in the avian skin. Science 357, 811–815. doi: 10.1126/science.aai7868
Silver, F. H., Horvath, I., and Foran, D. J. (2002). Mechanical implications of the domain structure of fiber-forming collagens: comparison of the molecular and fibrillar flexibilities of the alpha1-chains found in types I-III collagen. J. Theor. Biol. 216, 243–254. doi: 10.1006/jtbi.2002.2542
Steitz, S. A., Speer, M. Y., McKee, M. D., Liaw, L., Almeida, M., Yang, H., et al. (2002). Osteopontin inhibits mineral deposition and promotes regression of ectopic calcification. Am. J. Pathol. 161, 2035–2046. doi: 10.1016/S0002-9440(10)64482-3
Sun, Y., Chen, C. S., and Fu, J. (2012). Forcing stem cells to behave: a biophysical perspective of the cellular microenvironment. Annu. Rev. Biophys. 41, 519–542. doi: 10.1146/annurev-biophys-042910-155306
Suphasiriroj, W., Mikami, M., and Sato, S. (2013). Comparative studies on microvascular endothelial cells isolated from periodontal tissue. J. Periodontol. 84, 1002–1009. doi: 10.1902/jop.2012.120453
Tabata, S., Ozaki, H. S., Nakashima, M., Uemura, M., and Iwamoto, H. (1998). Innervation of blood vessels in the rat incisor pulp: a scanning electron microscopic and immunoelectron microscopic study. Anat. Rec. 251, 384–391. doi: 10.1002/(sici)1097-0185(199807)251:3<384::aid-ar14>3.0.co;2-k
Takebe, T., Enomura, M., Yoshizawa, E., Kimura, M., Koike, H., Ueno, Y., et al. (2015). Vascularized and complex organ buds from diverse tissues via mesenchymal cell-driven condensation. Cell Stem Cell. 16, 556–565. doi: 10.1016/j.stem.2015.03.004
Ten Cate, A. R. (1997). The development of the periodontium–a largely ectomesenchymally derived unit. Periodontol. 2000 13, 9–19. doi: 10.1111/j.1600-0757.1997.tb00093.x
Thesleff, I., Vaahtokari, A., Kettunen, P., and Aberg, T. (1995). Epithelial-mesenchymal signaling during tooth development. Connect. Tissue Res. 32, 9–15. doi: 10.3109/03008209509013700
Thesleff, I., Vaahtokari, A., Vainio, S., and Jowett, A. (1996). Molecular mechanisms of cell and tissue interactions during early tooth development. Anat. Rec. 245, 151–161. doi: 10.1002/(sici)1097-0185(199606)245:2<151::aid-ar4>3.0.co;2-#
Tomokiyo, A., Wada, N., and Maeda, H. (2019). Periodontal ligament stem cells: regenerative potency in periodontium. Stem Cells Dev. 28, 974–985. doi: 10.1089/scd.2019.0031
Tomokiyo, A., Yoshida, S., Hamano, S., Hasegawa, D., Sugii, H., and Maeda, H. (2018). Detection, characterization, and clinical application of mesenchymal stem cells in periodontal ligament tissue. Stem Cells Int. 2018:5450768. doi: 10.1155/2018/5450768
Trubiani, O., Pizzicannella, J., Caputi, S., Marchisio, M., Mazzon, E., Paganelli, R., et al. (2019). Periodontal ligament stem cells: current knowledge and future perspectives. Stem Cells Dev. 28, 995–1003. doi: 10.1089/scd.2019.0025
Vainio, S., and Thesleff, I. (1992). Sequential induction of syndecan, tenascin and cell proliferation associated with mesenchymal cell condensation during early tooth development. Differentiation 50, 97–105. doi: 10.1111/j.1432-0436.1992.tb00490.x
Vesela, B., Svandova, E., Bobek, J., Lesot, H., and Matalova, E. (2019). Osteogenic and angiogenic profiles of mandibular bone-forming cells. Front. Physiol. 10:124. doi: 10.3389/fphys.2019.00124
Volponi, A. A., and Sharpe, P. T. (2013). The tooth – a treasure chest of stem cells. Br. Dent. J. 215, 353–358. doi: 10.1038/sj.bdj.2013.959
Wada, N., Maeda, H., Hasegawa, D., Gronthos, S., Bartold, P. M., Menicanin, D., et al. (2014). Semaphorin 3A induces mesenchymal-stem-like properties in human periodontal, ligament cells. Stem Cells Dev. 23, 2225–2236. doi: 10.1089/scd.2013.0405
Wada, N., Menicanin, D., Shi, S., Bartold, P. M., and Gronthos, S. (2009). Immunomodulatory properties of human periodontal ligament stem cells. J. Cell. Physiol. 219, 667–676. doi: 10.1002/jcp.21710
Wang, L., Foster, B. L., Kram, V., Nociti, F. H. Jr., Zerfas, P. M., Tran, A. B., et al. (2014). Fibromodulin and biglycan modulate periodontium through TGFβ/BMP signaling. J. Dent. Res. 93, 780–787. doi: 10.1177/0022034514541126
Wang, Y., Wan, C., Deng, L., Liu, X., Cao, X., Gilbert, S. R., et al. (2007). The hypoxia-inducible factor alpha pathway couples angiogenesis to osteogenesis during skeletal development. J. Clin. Invest. 117, 1616–1626. doi: 10.1172/JCI31581
Wu, X., Shi, W., and Cao, X. (2007). Multiplicity of BMP signaling in skeletal development. Ann. N. Y. Acad. Sci. 1116, 29–49. doi: 10.1196/annals.1402.053
Xu, R. (2014). Semaphorin 3A: a new player in bone remodeling. Cell Adh. Migr. 8, 5–10. doi: 10.4161/cam.27752
Yamada, S., Tomoeda, M., Ozawa, Y., Yoneda, S., Terashima, Y., Ikezawa, K., et al. (2007). PLAP-1/asporin, a novel negative regulator of periodontal ligament mineralization. J. Biol. Chem. 282, 23070–23080. doi: 10.1074/jbc.M611181200
Yamamoto, N., Maeda, H., Tomokiyo, A., Fujii, S., Wada, N., Monnouchi, S., et al. (2012). Expression and effects of glial cell line-derived neurotrophic factor on periodontal ligament cells. J. Clin. Periodontol. 39, 556–564. doi: 10.1111/j.1600-051X.2012.01881.x
Yamaza, T., Ren, G., Akiyama, K., Chen, C., Shi, Y., and Shi, S. (2011). Mouse mandible contains distinctive mesenchymal stem cells. J. Dent. Res. 90, 317–324. doi: 10.1177/0022034510387796
Yang, N., Li, Y., Wang, G., Ding, Y., Jin, Y., and Xu, Y. (2017). Tumor necrosis factor-α suppresses adipogenic and osteogenic differentiation of human periodontal ligament stem cell by inhibiting miR-21/Spry1 functional axis. Differentiation 97, 33–43. doi: 10.1016/j.diff.2017.08.004
Yavropoulou, M. P., and Yovos, J. G. (2016). The molecular basis of bone mechanotransduction. J. Musculoskelet. Neuronal. Interact. 16, 221–236.
Yeasmin, S., Ceccarelli, J., Vigen, M., Carrion, B., Putnam, A. J., Tarle, S. A., et al. (2014). Stem cells derived from tooth periodontal ligament enhance functional angiogenesis by endothelial cells. Tissue Eng. Part A 20, 1188–1196. doi: 10.1089/ten.TEA.2013.0512
Yegutkin, G. G. (2008). Nucleotide- and nucleoside-converting ectoenzymes: important modulators of purinergic signalling cascade. Biochim. Biophys. Acta 1783, 673–694. doi: 10.1016/j.bbamcr.2008.01.024
Yen, A. H., and Sharpe, P. T. (2008). Stem cells and tooth tissue engineering. Cell Tissue Res. 331, 359–372. doi: 10.1007/s00441-007-0467-6
Yu, T., Volponi, A. A., Babb, R., An, Z., and Sharpe, P. T. (2015). Stem cells in tooth development, growth, repair, and regeneration. Curr. Top. Dev. Biol. 115, 187–212. doi: 10.1016/bs.ctdb.2015.07.010
Yuan, Y., and Chai, Y. (2019). Chapter Four - Regulatory mechanisms of jaw bone and tooth development. Curr. Top. Dev. Biol. 133, 91–118. doi: 10.1016/bs.ctdb.2018.12.013
Zhang, J., Kawashima, N., Suda, H., Nakano, Y., Takano, Y., and Azuma, M. (2006). The existence of CD11c+ sentinel and F4/80+ interstitial dendritic cells in dental pulp and their dynamics and functional properties. Int. Immunol. 18, 1375–1384. doi: 10.1093/intimm/dxl070
Zhao, Y., Bower, A. J., Graf, B. W., Boppart, M. D., and Boppart, S. A. (2013). Imaging and tracking of bone marrow-derived immune and stem cells. Methods Mol. Biol. 1052, 57–76. doi: 10.1007/7651_2013_28
Zhou, T., Pan, J., Wu, P., Huang, R., Du, W., Zhou, Y., et al. (2019). Dental follicle cells: roles in development and beyond. Stem Cells Int. 2019:9159605. doi: 10.1155/2019/9159605
Keywords: development, mouse, mandible, mesenchyme, condensation, odontogenesis, osteogenesis
Citation: Svandova E, Peterkova R, Matalova E and Lesot H (2020) Formation and Developmental Specification of the Odontogenic and Osteogenic Mesenchymes. Front. Cell Dev. Biol. 8:640. doi: 10.3389/fcell.2020.00640
Received: 05 May 2020; Accepted: 25 June 2020;
Published: 17 July 2020.
Edited by:
Rafaela Scariot, Universidade Positivo, BrazilReviewed by:
Rajprasad Loganathan, Johns Hopkins University, United StatesMilena Binhame Albini Martini, Universidade Positivo, Brazil
Suyany Gabriely Weiss, Universidade Positivo, Brazil
Copyright © 2020 Svandova, Peterkova, Matalova and Lesot. This is an open-access article distributed under the terms of the Creative Commons Attribution License (CC BY). The use, distribution or reproduction in other forums is permitted, provided the original author(s) and the copyright owner(s) are credited and that the original publication in this journal is cited, in accordance with accepted academic practice. No use, distribution or reproduction is permitted which does not comply with these terms.
*Correspondence: Herve Lesot, Herve.Lesot@gmail.com