- 1Institute of Experimental Botany of the Czech Academy of Sciences, Centre of the Region Haná for Biotechnological and Agricultural Research, Olomouc, Czechia
- 2Department of Cell Biology and Genetics, Faculty of Science, Palacký University Olomouc, Olomouc, Czechia
- 3Council for Agricultural Research and Economics (CREA) Research Centre for Genomics and Bioinformatics via San Protaso 302, Fiorenzuola d’Arda, Italy
- 4Department of Plant Developmental Genetics, Institute of Biophysics of the Czech Academy of Sciences, Brno, ;Czechia
- 5Department of Geoinformatics, Faculty of Science, Palacký University Olomouc, Olomouc, Czechia
- 6Department of Field Crops, Faculty of Agriculture, University of Çukurova, Adana, Turkey
- 7Council for Agricultural Research and Economics (CREA) Research Centre for Cereal and Industrial Crops, Foggia, Italy
Wild emmer wheat is an excellent reservoir of genetic variability that can be utilized to improve cultivated wheat to address the challenges of the expanding world population and climate change. Bearing this in mind, we have collected a panel of 263 wild emmer wheat (WEW) genotypes across the Fertile Crescent. The genotypes were grown in different locations and phenotyped for heading date. Genome-wide association mapping (GWAS) was carried out, and 16 SNPs were associated with the heading date. As the flowering time is controlled by photoperiod and vernalization, we sequenced the VRN1 gene, the most important of the vernalization response genes, to discover new alleles. Unlike most earlier attempts, which characterized known VRN1 alleles according to a partial promoter or intron sequences, we obtained full-length sequences of VRN-A1 and VRN-B1 genes in a panel of 95 wild emmer wheat from the Fertile Crescent and uncovered a significant sequence variation. Phylogenetic analysis of VRN-A1 and VRN-B1 haplotypes revealed their evolutionary relationships and geographic distribution in the Fertile Crescent region. The newly described alleles represent an attractive resource for durum and bread wheat improvement programs.
1 Introduction
Bread wheat (Triticum aestivum L.) and durum wheat (T. turgidum L. var. durum Desf.) are essential crops. Wheat has been the staple food of the major civilizations of Europe, West Asia, and North Africa for 8 000 years. The origin of these species is closely related to the development of human civilization. Although bread wheat is most successful between the latitudes of 30° and 60°N and 27° and 40°S (Nuttonson, 1955), durum wheat is grown mainly in the Mediterranean area (Royo et al., 2017). These species’ spread from their site of origin is connected with the ability to adapt to various climates and time their flowering to favorable conditions (Dubcovsky and Dvorak, 2007). This is controlled by the flowering pathway, consisting of photoperiod and vernalization pathways. While the photoperiodic pathway promotes flowering under long-day conditions, the vernalization pathway is induced by cold. Major vernalization genes are VERNALIZATION1 (VRN1), VERNALIZATION2 (VRN2), and VERNALIZATION3 (VRN3). VRN1, coding for a MADS-box type II transcription factor homologous to Arabidopsis APETALA1 (Yan et al., 2003), acts as a floral activator and is expressed in leaves and shoot apical meristem (Jin and Wei, 2016). VRN1 protein has a typical “MIKC” structure, formed by four domains: MADS (DNA binding), I (intervening; for dimerization), K (keratin-like; involved in dimer and tetramer formation), and C-terminal (carboxyl-terminal; variable, important for transactivation and higher-order complex formation) (Egea-Cortines et al., 1999; Saedler et al., 2001; Becker and Theißen, 2003; Lai et al., 2021). In wheat with winter growth habit, VRN1 alleles are intact, and their expression is induced by a period of cold treatment called vernalization (Trevaskis et al., 2003). Natural mutations of VRN1 promoter or intron 1 regions cause strong expression of the gene resulting in spring growth habit (Fu et al., 2005). In general, intron 1 mutations causing the spring growth habit span so-called ‘critical region’, which seems to comprise important regulatory site(s) (Fu et al., 2005), such as the RIP3 region, a binding site of the putative flowering repressor TaGRP2 (Xiao et al., 2014). It was shown that sequence variability of recessive vrn-A1 allele can affect its basal expression and the vernalization requirement. Hexaploid cultivar Jagger with the vrn-A1a allele requires three weeks of vernalization treatment whereas cultivar 2174 carrying the vrn-A1b allele containing exon 7 mutation needs six weeks (Li et al., 2013).
Wild emmer (T. turgidum ssp. dicoccoides) is an allotetraploid (2n=4x=28; genomes BBAA) species. Genome B was derived from an ancient species closely related to the extant Aegilops speltoides, while genome A was derived from T. urartu (Mayer et al., 2014). This wild wheat is easily crossed with durum and common wheat, yielding F1 hybrids, which are fully or partially fertile (Harlan and Wet, 1971). Pairing between the “wild” and “domesticated” chromosomes of the B and A genome is complete. Thus, almost all alleles can be transferred by simple plant breeding procedures from the “wild” into their “domesticated” homologues. Wild emmer is native to the Fertile Crescent, where it grows in two main regions: the Syria-Israeli and the Turkish-Iranian, where it occupies a variety of primary and secondary habitats (Maccaferri et al., 2019). Wild emmer wheat harbors rich allelic diversity for numerous important traits, including agronomic characteristics, grain quality, and resistance to biotic and abiotic stresses (Nevo et al., 2012; Fadida-Myers et al., 2022). A large number of genes and QTLs that are valuable for wheat improvement have been identified in the wild emmer gene pool and mapped (Reader and Miller, 1991; Peng et al., 2000; Rong et al., 2000; Chee et al., 2001; Peng et al., 2003; Merchuk-Ovnat et al., 2016; Balla et al., 2022a; Balla et al., 2022b).
Here, we present the heading time data for a panel of 263 wild emmer wheat genotypes collected in the Fertile Crescent and GWAS analysis of 178 winter genotypes from the panel. We have selected 95 genotypes with diverse growth habits and flowering times and sequenced their full VRN-A1 and VRN-B1 genes. We discovered several mutations in both coding and non-coding regions and examined the expression of seven selected genotypes. Identifying novel alleles causing early/late flowering could bring value to the breeding of improved genotypes.
2 Materials and methods
2.1 Plant material and growth conditions
2.1.1 Phenotypic evaluation in the field
A total number of 263 wild emmer wheat genotypes (T. dicoccoides; Supplementary Table 1) from the region of Fertile Crescent, including both spring and winter growth types (according to the GRIN-Global database (Version: 2.0.5.0) (https://npgsweb.ars-grin.gov/gringlobal/search)) was used for heading date experiments in Turkey (2016/2017, 2017/2018 and 2018/2019; Adana (37.015962, 35.355740)) and Italy (2016/2017; Fiorenzuola d’Arda (44.9276601, 9.8945892), 2017/2018 and 2018/2019; Foggia (41.460391, 15.501311)). In addition growth habit was retrieved for most of the accessions from passport data provided by Genebanks. First, seeds were germinated in pots under controlled conditions in the greenhouse (long day, 16 h of light at 20°C and 8 h of darkness at 16°C). At the third-leaf stage, seedlings were transferred to the field and planted in the 1m row (seven plants from the same genotype in one row), which was done in two replicates for each genotype. When the spike emerged in 75% of the plants in the row, the day was fixed as the heading date.
2.1.2 DNA extraction for VRN1 sequencing
We selected 95 wild emmer wheat genotypes to cover early to late flowering genotypes (Supplementary Table 2). T. dicoccoides line Tabigha 15 for sequencing of the Vrn-A1d allele was provided by The Institute of Evolution Wild Cereal Gene Bank at the University of Haifa. Seeds were imbibed on Petri dishes at 21°C for 48 h and then placed at 4°C for two days to synchronize germination. Seedlings were transferred to a pot and placed in the growth room under long-day conditions (16 h of light at 20°C and 8 h of darkness at 16°C). For DNA isolation, leaves were collected three weeks after potting.
2.2 SNP genotyping
The DNA was extracted from 8–10 days-old seedlings (4-5 plants pooled) following the CTAB method. After standard Axiom sample preparation, all accessions were genotyped with the 35 K Wheat Breeders Affymetrix-SNP array (Axiom Wheat Breeder’s Genotyping Array). Allele calling was carried out using the Affymetrix proprietary software package GTC, following the Axiom® Best Practices Genotyping Workflow. In addition, all genotypes were genotyped for the Ppd-A1 allele, as reported in Beales et al. (2007).
2.3 Genome-wide association study
The Genome-Wide Association Study (GWAS) for heading time in winter genotypes was run by GAPIT version 3 using a fixed and random model circulating probability unification (FarmCPU) (Liu et al., 2016), and the Bayesian information and Linkage-disequilibrium Iteratively Nested Keyway (BLINK) model (Huang et al., 2019). The GWAS for all data obtained from different locations and years was done from BLUP values (Best Linear Unbiased Predictions) which can eliminate the environmental and year deviations. BLUP values were counted with R package Phenotype (Piepho et al., 2008). Since the SNP frequency is not too high in T. dicoccoides genome to perform linkage disequilibrium analysis (most of the SNPs were alone in blocks; data not shown), the candidate genes were searched 500kb upstream and downstream from the SNP position. The protein sequences of candidate genes were extracted from T. dicoccoides genome (https://plants.ensembl.org/Triticum_dicoccoides/Info/Index) and annotated based on the best UniProtKB/Swiss-Prot blastp hit online at https://blast.ncbi.nlm.nih.gov/. The panel of 178 winter genotypes used for GWAS, including their heading time data and BLUP values, is listed in Supplementary Table 3.
2.4 PCR amplification and sequencing
Genomic DNA for sequencing was extracted from leaves of two-week-old plants using NucleoSpin Plant II (MACHEREY-NAGEL, Germany) according to the manufacturer’s instructions.
Sequences of VRN1 genes were obtained by sequencing overlapping PCR products using several sequencing protocols as described in (Strejčková et al., 2021). Briefly, short PCR products (< 1200 bp) were sequenced by the Sanger method, while long PCR products (> 2700 bp) were sequenced with the Illumina iSeq platform.
2.4.1 PCR amplification
DNA was amplified using Bio-Rad C1000 Touch Thermal Cycler (Bio–Rad, USA). Primers and conditions used for PCR are listed in Supplementary Table 4.
2.4.2 Sanger sequencing
PCR clean-up was performed by ExoSap (ThermoFisher Scientific, USA). The sequencing reactions were performed using the BigDye1 Terminator v3.1 Cycle Sequencing Kit (Applied Biosystems, USA) and purified using the Agencourt Clean SEQ Dye-Terminator Removal kit (Beckmann Coulter, USA). The reactions were analyzed on the ABI3730xl DNA analyzer (Applied Biosystems, USA). The resultant sequences were trimmed and assembled using Geneious Prime® 2022.0.1 (http://www.geneious.com). Assemblies were verified by alignment with the reference VRN1 sequence of Triple Dirk C (TDC) (Genbank accessions AY747600.1 and AY747604.1).
2.4.3 Illumina sequencing
PCR amplicons were purified using AMPure XP Beads (Beckman Coulter, USA) with a DNA volume/beads ratio of 1:1. DNA was quantified using the Qubit dsDNA HS assay system (Invitrogen, USA). For each PCR amplicon or pool of amplicons, a sequencing library was prepared using the NEBNext® Ultra™ II DNA Library Prep Kit for Illumina® with the following modifications: (i) DNA was fragmented in 50 µL solution using a Bioruptor Plus (Diagenode, Belgium) eight times for 30 s on the HIGH setting; (ii) size selection was performed for an approximate insert size of 500–700 bp; and (iii) PCR enrichment was carried out in 3–4 cycles. Libraries were equimolarly pooled and sequenced on an Illumina iSeq system with 150 bp paired-end (PE) reads to achieve a minimal amplicon coverage of 100×.
2.5 Sequencing data analysis
Read trimming based on quality (Q30) and sequencing adaptor removal were performed with Trimmomatic (v.0.32) (Bolger et al., 2014). All trimmed reads for each sample were mapped to the VRN1 TDC reference with BWA-MEM (v.0.7.15) (Li, 2013). Mapped reads for each genome variant (A and B) were extracted from the bam file by SAMtools (v.1.9) (Li et al., 2009) and de novo assembled by Spades (v.3.13.0) (Bankevich et al., 2012). Mapping results were manually reviewed with the Integrative Genome Viewer v.2.6.3 (IGV) (Robinson et al., 2017). Obtained sequences were further analyzed in Geneious Prime® 2022.0.1 (http://www.geneious.com).
2.6 In silico protein analysis
VRN1 protein domains were identified using the InterPro database (Mulder et al., 2007). The secondary structure of proteins was predicted using the module EMBOSS Protein Analysis version:1.0 (Rice et al., 2000) in the Geneious Prime® 2022.0.1. Protein alignments were done in the Geneious Prime® 2022.0.1 using MAFFT v7.490 (Katoh et al., 2002; Katoh and Standley, 2013).
2.7 Phylogenetic analysis and maps
Nucleotide alignments for phylogenetic analysis were conducted in Geneious Prime® 2022.0.1 (http://www.geneious.com) by MAFFT v7.490 (Katoh et al., 2002; Katoh and Standley, 2013). The evolutionary history was inferred using the Neighbor Joining tree build method (Saitou and Nei, 1987) and Tamura-Nei genetic distance method (Tamura and Nei, 1993). The bootstrap consensus tree is inferred from 1000 replicates. Data preparation for map-making was done using Microsoft Excel. The maps were created using ArcGIS Pro 3.0.2 https://www.esri.com/en-us/arcgis/products/arcgis-pro/resources.
2.8 RNA extraction and gene expression analysis
We selected seven genotypes (PI 538660, PI 538646, PI 466935, PI 471057, PI 466991, PI 428082, and PI 487263) with mutated alleles and the hexaploid cultivar Triple Dirk C (TDC) to analyze VRN-1 transcription. Seeds were imbibed on Petri dishes and placed at 4°C for three days to synchronize germination. Six seedlings of each variety were transferred to pots and placed in a growth chamber under LD conditions for two weeks). Afterward, they were vernalized for four weeks (8 h of light at 6°C and 16 h of darkness at 6°C). Finally, they were returned to the growth chamber under LD conditions. We collected leaves two, four, six, and eight weeks after potting. Total RNA was extracted using a Quick-RNA Miniprep Kit (Zymo Research, USA), including DNAse treatment according to the manufacturer’s instructions. cDNA was synthesized using a RevertAid First Strand cDNA Synthesis Kit (Thermo Scientific™, USA) according to the manufacturer’s instructions using 2 μg of total RNA and anchored-oligo (dT)18 primers. The gene expression level was determined using reverse transcription-qPCR (RT–qPCR). RT–qPCR was performed using qPCR 2x SYBR Master Mix (Top-Bio, Czech Republic) on the CFX96TM Real-Time PCR Detection System (Bio–Rad, USA). The data were analyzed using the 2-ΔΔCq method with CFX Maestro 2.0 software (Bio–Rad, USA). Three biological replicate PCR amplifications were performed for each sample. The expression level was standardized against the reference glyceraldehyde-3-phosphate dehydrogenase (GAPDH), according to Ivaničová et al. (2017). Sequences of all primers used for RT–qPCR are listed in Supplementary Table 5. We also scored heading time when half of the first spike had emerged.
2.9 Statistical analysis
The statistical significance of expression data was determined by one-way analysis of variance (ANOVA), followed by post-hoc Duncan’s Multiple Range test (α=0,05). ANOVA was performed in Statistica 14.0.0.5 (Statsoft).
3 Results
3.1 Heading time differences in the collection of wild emmer wheat
Phenotypic data were obtained for the collection of 286 WEWs from the Fertile Crescent. All genotypes carry the photoperiod-sensitive Ppd-A1 allele. The heading date (HD) was scored in three consecutive years in two different countries - Turkey (TUR) (Supplementary Table 6) and Italy (IT) (Supplementary Table 7). Mean heading time ranged from 124 to 156 days (TUR) and from 143 to 170 days (IT).
Within the large WEW collection evaluated for HD, a panel of 178 genotypes with winter growth habit was selected to be used in GWAS analysis based on 12,101 polymorphic SNPs from the Axiom 35K wheat breeder array. In the GWAS for heading date (density histograms for observed phenotypic data are presented in Supplementary Figure 1), sixteen highly significant marker-trait associations were identified by GAPIT (seven by FarmCPU and nine by BLINK model). They were located on chromosomes 1B (1), 3A (2 and 3), 3B (4 and 5), 4A (6), 5A (7 and 8), 5B (9), 6A (10), 6B (11), 7B (12, 13, and 14) and ND (not determined, two SNPs), explaining 2% to 36% of the phenotypic variations. The allelic effect for SNP markers associated with the heading date is shown in Supplementary Figure 2. The details of these markers are summarized in Supplementary Table 8 and depicted as Manhattan and QQ plots in Figure 1. Candidate genes are listed in Supplementary Table 9. Out of the 153 candidate genes, 122 were annotated based on the UniProtKB/Swiss-Prot blastp hit. Some of the orthologs were reported to have a function in the flowering regulation of other species or are associated with the epigenetic regulation of plant development. None of the MTA is coincident with known VRN loci because of the array’s SNP coverage.
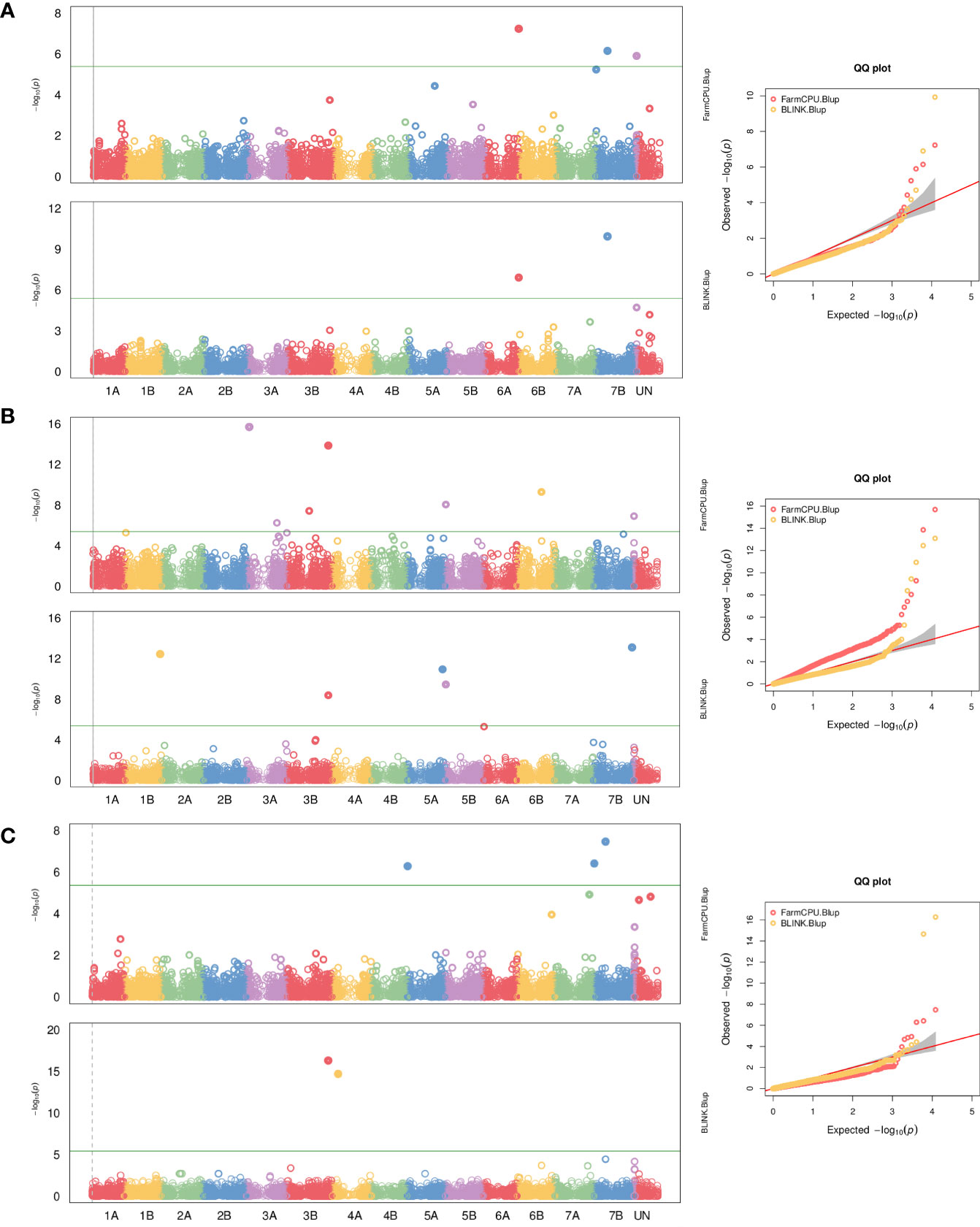
Figure 1 Genome-wide association study (GWAS) result plots for heading date in the wild emmer wheat population. Manhattan and Quantile-quantile plots for BLINK and FarmCPU models for data from Italy (A), Turkey (B), and for both localities (C). The significant threshold (p=0.05) is indicated by the green horizontal line.
3.2 VRN1 sequence variability in a subset of 95 wild emmer wheat
We selected 95 WEW from the phenotyped panel to inspect the allelic diversity of the important flowering time regulator, the VRN1 gene, in both spring and early to late winter genotypes. Forty-one of the 95 selected genotypes are from Turkey, 29 from Israel, three from Syria, ten from Palestina, and 12 from Lebanon. The mean heading time of these genotypes ranges from 124 to 156 days when grown in Turkey and 143 to 170 days in Italy.
The full-length gene sequencing showed a significant variability within promoters, introns, and exons of both VRN-A1 and VRN-B1 alleles (Supplementary Material). Often, more mutations were identified within one allele, and in a number of genotypes were mutated both VRN1 homoeoalleles. Newly found mutations of promoter and introns or their combinations are depicted in Figures 2 and 3. Exonic mutations are summarized in Tables 1 and 2, and resulting changes in VRN1 proteins are illustrated in Figure 4. The positions of all mutations are given from the start codon. The minus (-) sign means upstream direction.
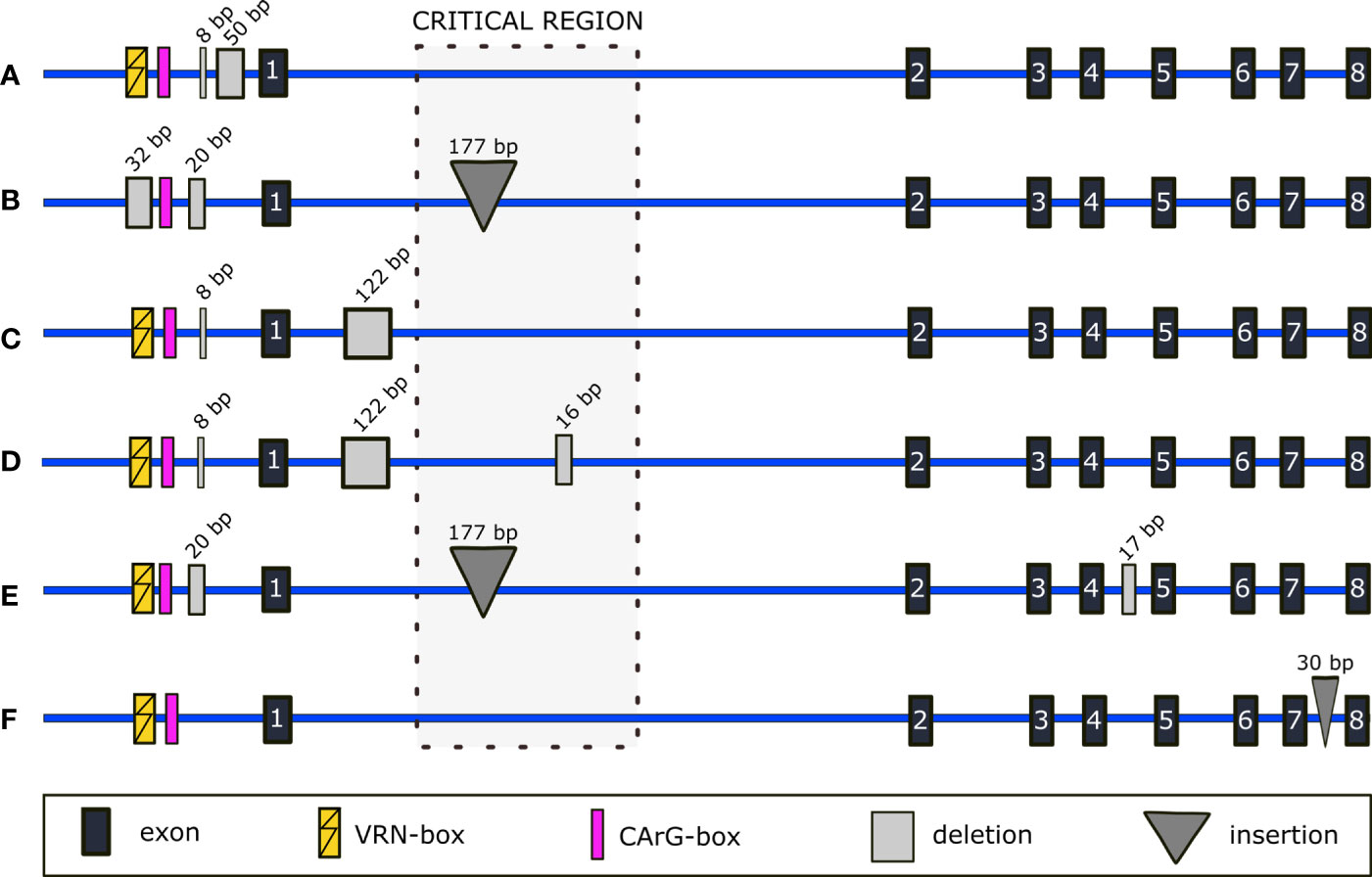
Figure 2 Schematic representation of selected indels detected within non-coding regions of VRN-A1. Promoter mutations in (A) correspond to known mutations of Vrn-A1f and Vrn-A1f-like alleles, but no intronic mutations are present. Mutations in (B) correspond to the Vrn-A1d allele with revealed 177-bp insertion in intron 1. Newly detected intron 1 deletion is shown in (C). In (D), known mutations of the VRN-A1b allele and novel 17-bp deletion of intron 4 are depicted. A novel deletion in intron 7 is represented in (E). An insertion of 30 bp situated in intron 7 is shown in (F).
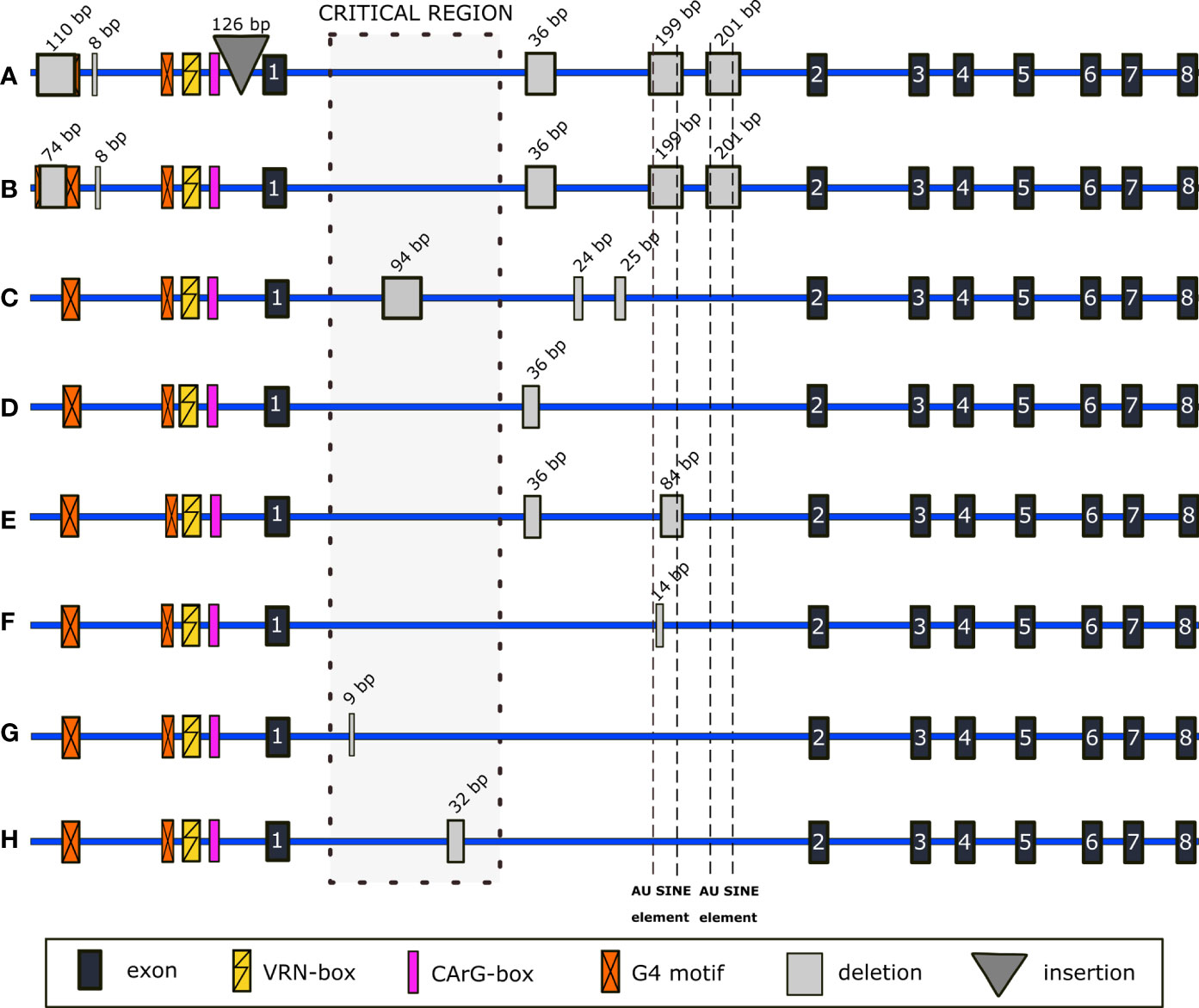
Figure 3 Schematic representation of selected indels detected within non-coding regions of VRN-B1. In (A) and (C–H), alleles with several different new indels are shown. In (B), promoter mutations correspond to mutations described for the Vrn-G1a allele (GenBank KX344118.1) and are combined with newly found mutations of intron 1.
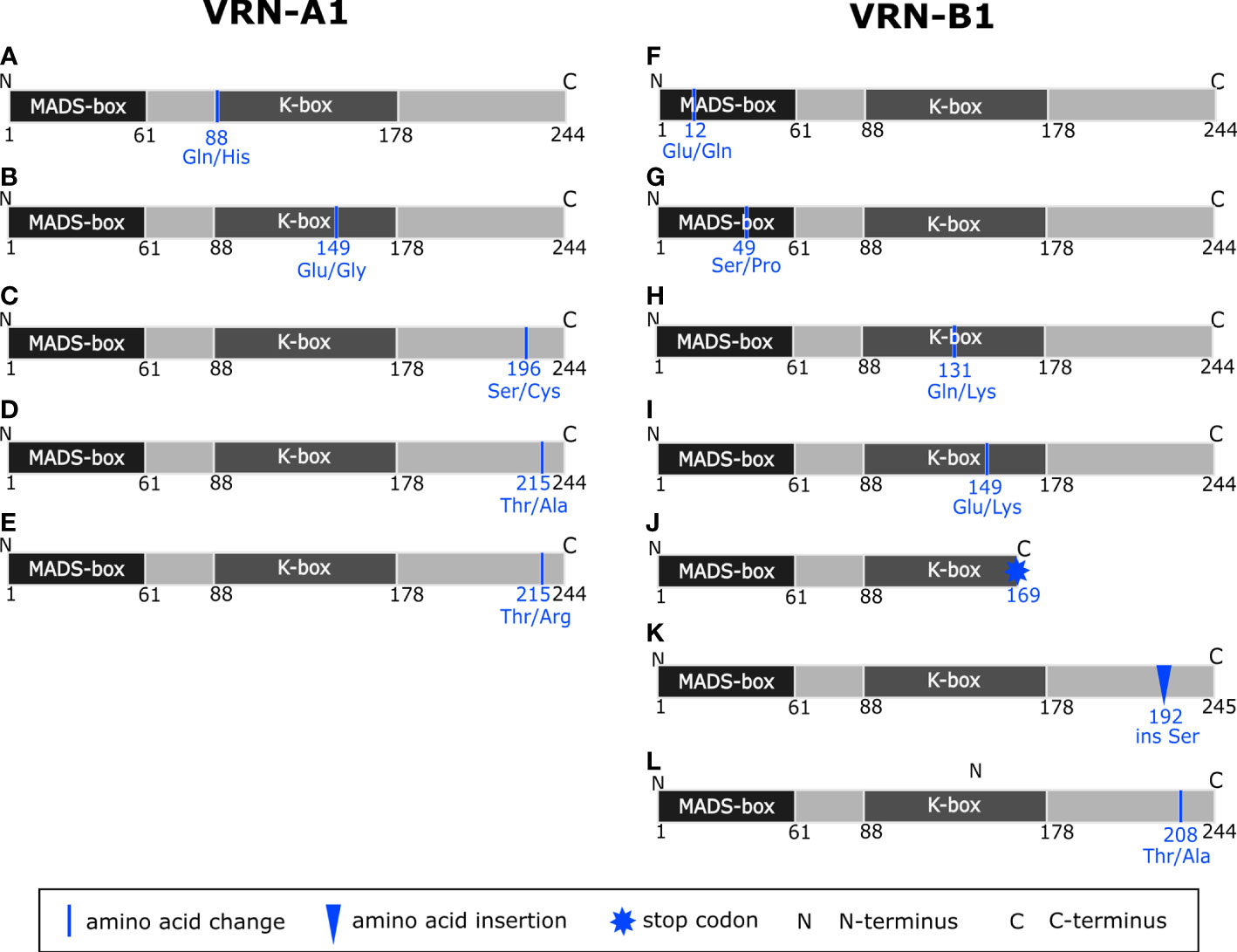
Figure 4 Schematic representation of predicted variants of VRN-A1 (A–E) and VRN-B1 (F–L) proteins based on the change in the nucleotide sequence of VRN-A1 and VRN-B1 genes, respectively. Domains were identified using the InterPro database (Mulder et al., 2007).
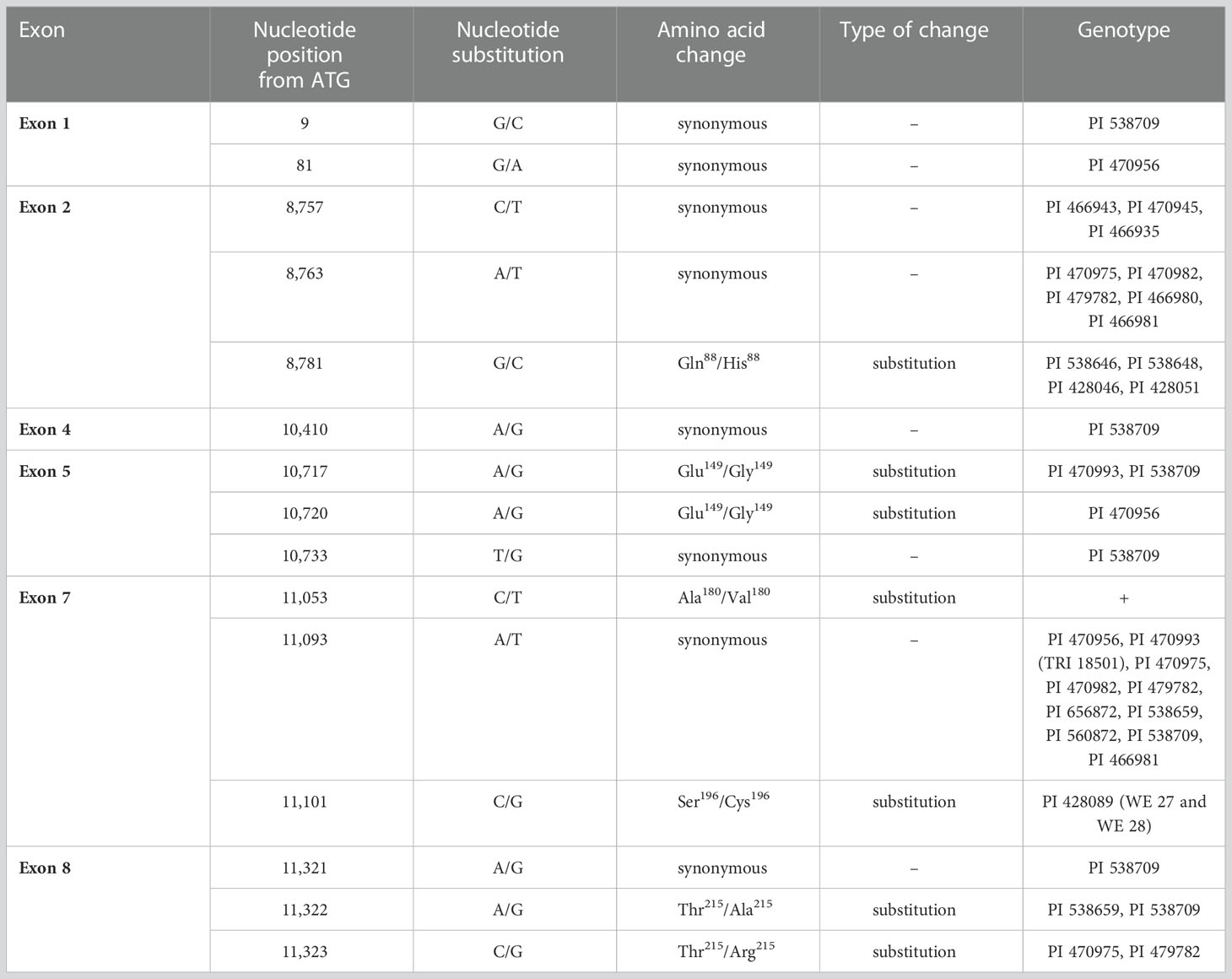
Table 1 Mutations within VRN-A1 exons. ´+´ group contains large number of genotypes (38) listed in the Supplementary Table 13.
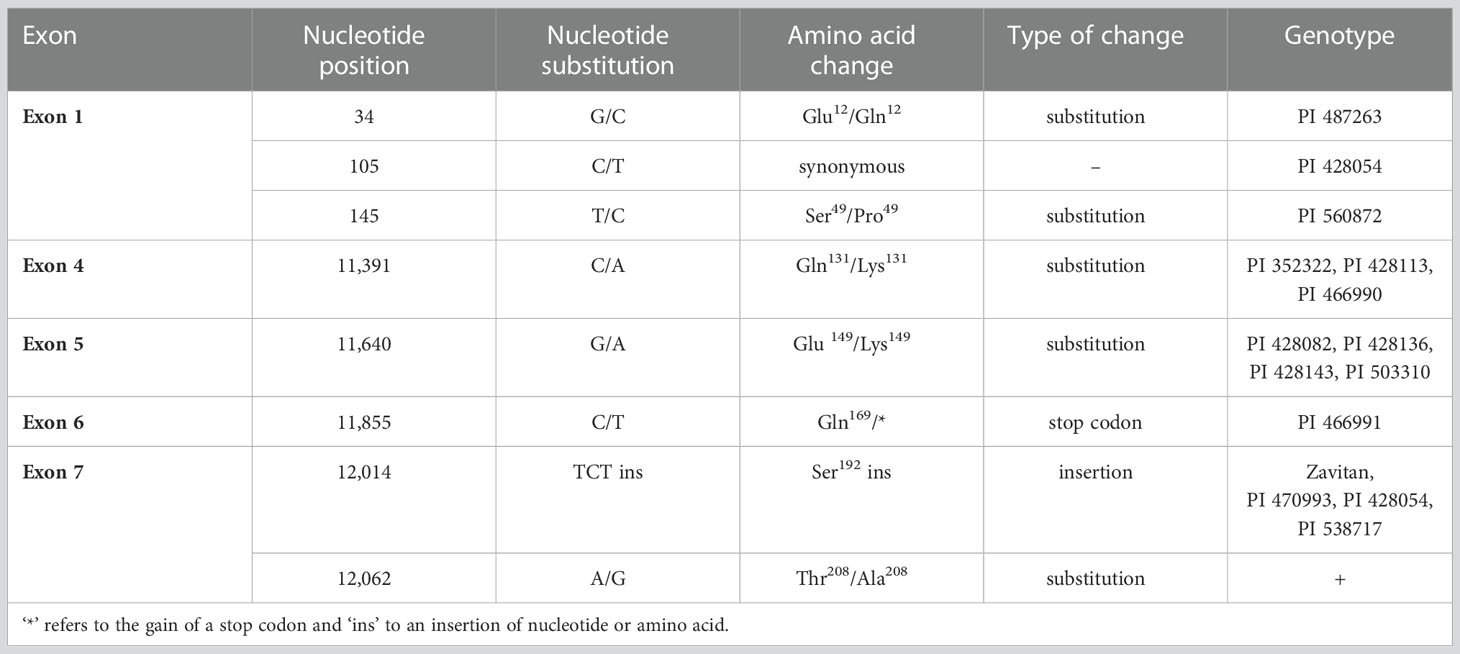
Table 2 Mutations within VRN-B1 exons. ´+´ group contains large number of genotypes (18) listed in the Supplementary Table 16.
3.3 Sequence variability of VRN-A1
3.3.1 Mutations of the promoter
Twenty-three wild emmer wheat genotypes (Supplementary Table 10) carry the VRN-A1b allele (Yan et al., 2004). They can be further divided according to the mutations in the VRN-box (Muterko et al., 2016) into Vrn-A1b.2 and vrn-A1b.4.
The known deletion of 8 bp at the -120 bp from the start codon (Golovnina et al., 2010) was found in seven winter and three spring genotypes (Supplementary Table 11).
The promoter of PI 560872 carries a combination of deletions (Figure 2A) described in Vrn-A1f (GenBank GQ451751) and Vrn-A1f-like (GenBank KT696537) alleles (Golovnina et al., 2010; Ivaničová et al., 2016). For a more detailed comparison of the three alleles, see Supplementary Table 12.
The winter genotype PI 428054 carries promoter mutations (GenBank OP831152) corresponding to the Vrn-A1d allele (Figure 2B).
3.3.2 Mutations within introns
As expected, we found most of the sequence variability in the intron 1. Besides SNPs, larger mutations were present in some samples. The 177-bp insertion (Strejčková et al., 2021) was detected in all genotypes with the VRN-A1b allele and in the genotype PI 428054 with the promoter mutation known from the Vrn-A1d allele. We sequenced Vrn-A1d from Israeli T. dicoccoides line Tabigha 15 (provided by The Institute of Evolution Wild Cereal Gene Bank at the University of Haifa), which was characterized only at the promoter sequence to date (Yan et al., 2004). Indeed, the Vrn-A1d allele from Tabigha 15 also contains the 177-bp insertion in the intron 1 (GenBank OP831151), as detected in PI 428054.
A newly found 122-bp deletion in the intron 1 (Figures 2C, D) was present only in genotypes carrying allele with an 8-bp deletion in the promoter and A11093T SNP in exon 7 (Ser196/Cys196). A 16-bp deletion was found in winter genotypes PI 470956 and PI 470993 (TRI 18501) (Figure 2D). Most of the genotypes possess the RIP3 1_SNP haplotype GGACC (Kippes et al., 2018), besides PI 466989 carrying RIP3 3_SNP haplotype CGACT and PI 538709 with the canonical RIP3 motif GGATC (Kippes et al., 2018).
A 17-bp deletion in the intron 4 of the VRN-A1b allele was found in four spring genotypes (PI 538698, PI 428015 (TRI 18481), PI 538697, and PI 538696) (Figure 2E).
We found a 30-bp insertion in the intron 7 of three winter genotypes (PI 466958, PI 538657, and PI 428055). This insertion was a duplication of an adjacent downstream sequence (Figure 2F).
3.3.3 Mutations involving coding sequence
Surprisingly, eight synonymous and seven non-synonymous SNPs were found within the exons 1, 2, 4, 5, 7, and 8 (Table 1 and Figure 4). Only non-synonymous SNPs will be described further in the text. Synonymous SNPs are summarized in Table 1.
In exon 2, we detected one non-synonymous SNP G8781C changing the amino acid (AA) from glutamine into histidine (Gln88/His88) in the K-box of VRN-A1 protein (Figure 4A). This SNP was detected exclusively in four winter late-flowering genotypes.
In exon 5, A10717G and A10720G SNPs causing the same amino acid substitution from glutamic acid to glycine (Glu149/Gly149) in the K-box (Figure 4B) were found. The first SNPs were detected in two and one winter genotype, respectively.
C11053T SNP in exon 7 (Ala180/Val180) (Díaz et al., 2012) was present in 38 winter genotypes (Supplementary Table 13). This allele is referred to as vrn-A1b and is associated with higher vernalization requirement and later flowering (Li et al., 2013). The so far unpublished non-synonymous SNP C11101G resulting in a change of serine into cysteine (Ser196/Cys196) in the C-terminal of the VRN-A1 protein (Figure 4C) was revealed in two winter genotypes in combination with Ala180/Val180.
Two non-synonymous SNPs; A11322G (Thr215/Ala215) and C11323 (Thr215/Arg215) affecting same amino acid of the C-terminus (Figures 4D, E), were detected in exon 8. Mutations of exon 8 were rare in our analyzed set of wild emmer wheat, emerging in four winter genotypes only.
All amino acid substitutions also change the predicted secondary structure of the VRN-A1 protein (Supplementary Figure 3A).
3.4 Sequence variability of VRN-B1
3.4.1 Mutations of the promoter
In 35 genotypes, we found SNP in the 23-bp G4 motif situated at -274 bp (Supplementary Table 14). PI 560872 and PI 656872 genotypes carried a 110-bp (spanning across the G4 motif, position -735 bp) and 8-bp deletion (-583 bp), together with 126-bp insertion (-100 bp) (Figure 3A) (GenBank OP831155). Golovnina et al. (2010) reported an identical transposon insertion at -100 bp (GenBank GQ451771.1). In PI 538659, promoter deletions identical to that described for Vrn-G1a (GenBank KX344118.1) (Shcherban et al., 2016) were detected (Figure 3B). Interestingly, the G4 motif is reconstituted by the adjacent two bp (Supplementary Figure 4). Contrary to its VRN-A1 homoeoallele, there were no mutations within the VRN- and CArG-box.
3.4.2 Mutations within introns
VRN-B1 intron 1 shows large sequence variability, including deletions of various lengths. In spring genotypes PI 538691 and PI 538695, the combination of 94-bp, 24-bp, and 25-bp deletions was detected (Figure 3C) (GenBank OP831153 and OP831154). We detected different deletions covering the Au SINE elements of VRN-B1 intron 1: complete 199- and partial 84- and 14-bp deletions of the first Au SINE and complete 201-bp deletion of the second one. Opposite to the complete deletions, partial deletions were found across a wide range of winter genotypes, from early to late flowering ones, as well as in spring. Several genotypes share a 36-bp deletion (Figure 3D) (PI 467001), mainly together with an 84-bp deletion (Figure 3E) (PI 428082, PI 352322, PI 428113, PI 428136 (TRI 18489), PI 428143, CItr 17675, PI 503310 and PI 466990), or 199- and 201-bp deletions (Figures 3A, E) (PI 656872, PI 538659 and PI 560872) spanning over the Au SINE elements. Another common mutation of intron 1 found in six out of 95 sequenced genotypes was the deletion of 14 bp located in the Au SINE element (Figure 3F and Supplementary Table 15). A 9-bp deletion was found only in the winter genotype PI 466961 (Figure 3G) and a 32-bp deletion in the winter genotype PI 428043 (Figure 3H).
3.4.3 Mutations involving coding sequence
In exons 1, 4, 5, 6, and 7 (Table 2 and Figure 4) of VRN-B1, seven different non-synonymous and one synonymous SNP were found. Only non-synonymous SNPs will be described in the text below. Synonymous SNPs are summarized in Table 2.
In exon 1 were detected the G34C SNP (MADS-box, Glu12/Gln12) (Figure 4F) and the T145C SNP, resulting in the Ser49/Pro49 change in the MADS-box of VRN-B1 protein (Figure 4G). Each of the mentioned SNPs was unique.
In exon 4 of two winter and one spring genotypes, C11391A SNP was found, causing AA substitution Gln131/Lys131 in the K-box of VRN-B1 (Figure 4H).
Another SNP, G11640A, causing AA substitution within the K-box (Glu149/Lys149), was detected in exon 5 of four winter genotypes (Figure 4I).
An interesting mutation resulting in premature stop codon was found in exon 6 (C11855T) of PI 466991. The predicted VRN-B1 protein is only 169 AA long and contains an intact MADS-box and partial K-box (Figure 4J).
Insertion of TCT into exon 7, causing insertion of Ser192 in the VRN-B1 protein, is present in four winter genotypes (Figure 4K). AA change Thr208/Ala208 encoded by a mutation in exon 7 (A12062G) was found exclusively in 13 early flowering winter genotypes and five spring genotypes (Supplementary Table 16 and Figure 4L).
All amino acid substitutions, except Lys131/Gln131 in the K-box, change the predicted secondary structure of the VRN-B1 protein (Supplementary Figure 3B).
3.5 Gene expression of selected VRN-A1 and VRN-B1 alleles
Six different genotypes (PI 428082, PI 466935, PI 466991, PI 471057, PI 487263, PI 538646, PI 538660) were chosen for the RT-qPCR experiment to assess the influence of some of the detected VRN1 mutations. We compared those genotypes with the bread wheat cultivar TDC and the late flowering PI 538660. TDC has the intact recessive VRN1 alleles, while PI 538660 possesses the vrn-A1b allele (C11053T SNP, Ala180/Val180) and intact vrn-B1 (Figure 5). The vrn-A1b allele showed to be the most abundant recessive allele in the sequenced panel.
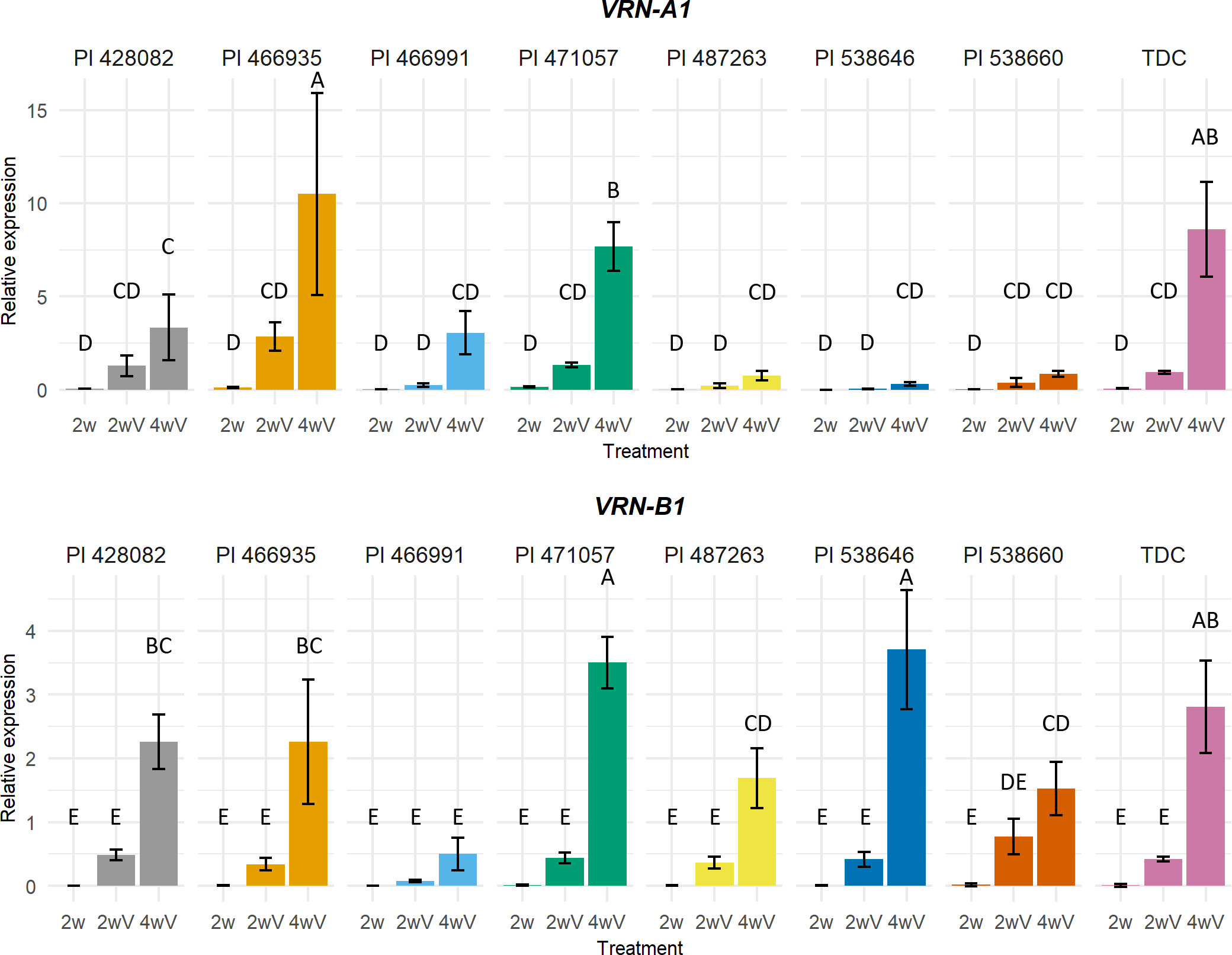
Figure 5 VRN-A1 and VRN-B1 expression profiles of PI 428082, PI 466935, PI 466991, PI 471057, PI 487263, PI 538646, PI 538660, and TDC. Expression data were analysed using one-way ANOVA with Duncan’s post-hoc test at α=0.05. Means that do not share a letter are statistically different. 2w – two weeks under 21°C LD, 2wV - two weeks under 21°C LD, followed by two weeks of vernalization, 4wV - two weeks under 21°C LD, followed by four weeks of vernalization.
After four weeks of vernalization treatment, the highest VRN-A1 expression was detected in the early flowering winter genotype PI 466935 with synonymous C8757T mutation in exon 2. On the contrary, the expression of late flowering PI 538646 with Gln88/His88 substitution in VRN-A1 K-box was very low. It was similar to PI 538660 and PI 487263, carrying the vrn-A1b allele for a stronger vernalization requirement. The expression level of PI 471057 intact vrn-A1 was similar to TDC, while in PI 428082 intact vrn-A1 showed lower expression compared to TDC.
The vrn-B1 allele from PI 466935 and the vrn-B1 allele from PI 428082 have the same expression profile. The vrn-B1 allele in PI 466935 has a small 6-bp deletion in intron 7. The vrn-B1 allele in PI 428082 carries intron 1 deletions and substitution in the K-box. The expression of PI 466991 vrn-B1 containing nonsense mutation was minimal. PI 471057 vrn-B1 with C-terminal substitution and PI 538646 vrn-B1 with 7-bp intron 1 insertion (expansion of microsatellite repeat ACCCCCC) expression was the highest. PI 487263 vrn-B1 with MADS-box substitution reached the same level as intact PI 538660 vrn-B1.
3.6 Distribution of VRN1 variants across the Fertile Crescent
Subsequently, we inspected the distribution of identified VRN1 variants across the Fertile Crescent. Because of the high sequence diversity across the sequenced panel, we decided to construct a phylogenetic tree for both VRN-A1 and VRN-B1 sequences (Supplementary Figures 5 and 6). We used resultant individual clades (further referred to as ‘group’) and mapped individuals from each group to their collection site to illustrate the distribution of VRN1 alleles (Figures 6 and 7).
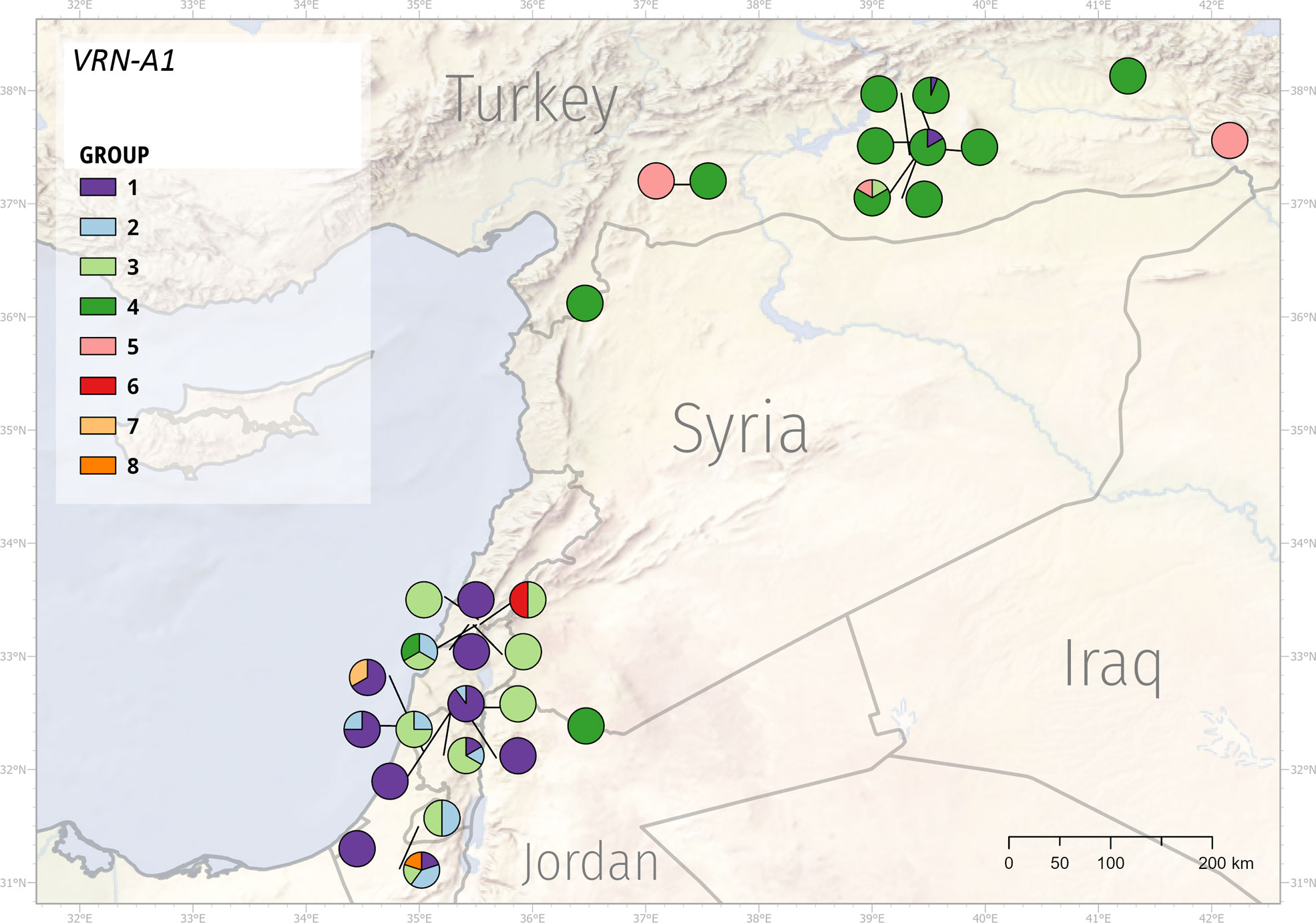
Figure 6 Geographic distribution of the VRN-A1 alleles. WEW genotypes formed eight groups according to the VRN-A1 mutations.
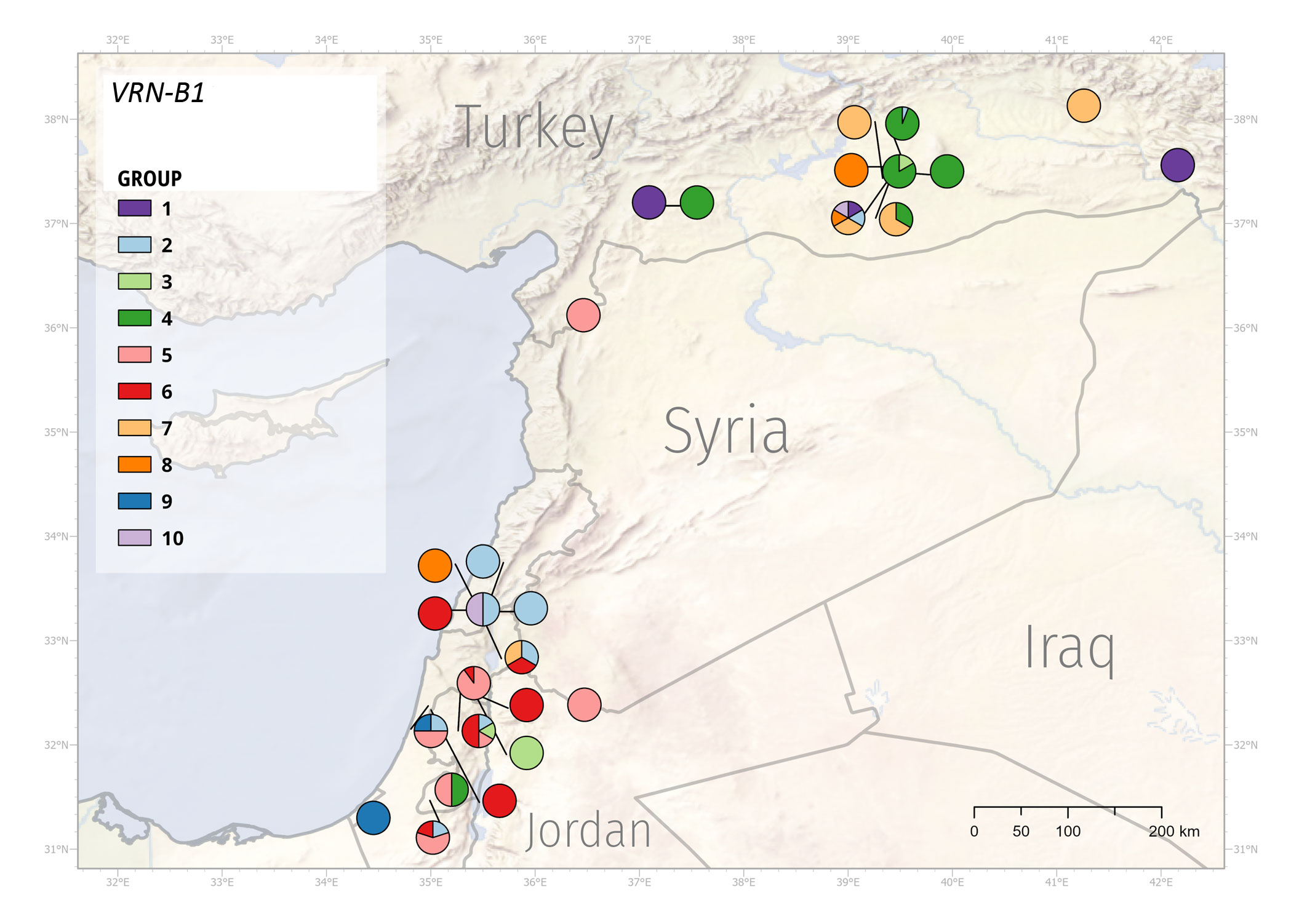
Figure 7 Geographic distribution of the VRN-B1 alleles. WEW genotypes formed 10 groups according to the VRN-B1 mutations.
Based on the VRN-A1 sequences, genotypes were split into eight groups (Supplementary Table 17). We could not obtain the full-length VRN-A1 sequence of PI 466988 and PI 538691. Therefore, we did not include these samples in the phylogenetic analysis. However, based on their partial sequences and genotyping, they most likely belong to groups 1 and 2, respectively. Group 1 contains all 23 genotypes with the VRN-A1b allele and one genotype with the Vrn-A1d allele. Group 2 includes nine genotypes with 122-bp deletions in intron 1 with combinations of two different mutations in exon 2, and 7. Group 3 contains alleles with high sequence similarity to the intact vrn-A1 allele. Group 4 is the most numerous one and covers all genotypes (38) with the exon7 C11053T SNP. This SNP results in amino acid change Ala180/Val180 associated with stronger vernalization requirement (Díaz et al., 2012; Li et al., 2013). Out of the 38 genotypes, 35 come from Turkey. Group 5 is formed by three genotypes from Turkey containing the A11093T SNP in exon 7. Groups 6-8 contain only one genotype each and cover genotypes with a very high number of SNPs, Vrn-A1c allele, and RIP3_3SNPs haplotype, respectively.
VRN-B1 sequence similarity divided genotypes into ten groups (Supplementary Table 18). Genotypes in group 1 share 36-, 199- and 201-bp deletions of intron 1. Group 2 genotypes possess 36- and 84-bp deletion in intron 1, besides PI 467001 carrying only 36-bp deletion. Genotypes in group 3 share 3-bp insertion in exon 7. Group 4 harbors 25 genotypes with intact vrn-B1 and PI 428043 with a 32-bp deletion in intron 1. Group 5 contains all 18 genotypes with A12062G SNP in exon 7, PI 487260, and PI 487263. Group 6 is very diverse, with 15 genotypes. Group 7 includes eight genotypes with intact vrn-B1. Group 8 contains only three genotypes, and groups 9 and 10 contain two genotypes each.
4 Discussion
Tetraploid durum wheat (T. turgidum L. ssp. durum (Desf.) Husn.) and hexaploid bread wheat (T. aestivum L.) are important crops grown on 220 million hectares, which is more than any other food crop (Foreign Agricultural Service/USDA, 2022). Both wheats share a common progenitor, wild emmer wheat (T. turgidum ssp. dicoccoides (Schrank ex Schübl.). Following the domestication in the Fertile Crescent, wheat evolved along ancient human migration roads and adapted to a wide range of agro-climatic regions (Balfourier et al., 2019). This was made possible by controlling the flowering time depending on the growing conditions. The flowering time is influenced by photoperiod and temperature. Wheat genotypes can be classified as winter, facultative or spring based on the requirement of a cold period to switch from the vegetative to the reproductive phase (vernalization). We studied a collection of 263 winter and spring WEWs from Turkey, Lebanon, Syria, Palestina, Israel, Iraq, and Iran, covering the Fertile Crescent region. In this collection, we observed heading time differences and identified alleles lost in modern wheat during the breeding process. Such alleles might be beneficial in breeding programs.
4.1 HD loci in a winter wild emmer population
We performed GWAS on 178 WEW genotypes and found 16 MTAs (Marker-trait associations) associated with heading time (Supplementary Table 7) The candidate genes were annotated based on the best Uniprot balstp hit (Supplementary Material 1).
Three candidate genes for AX-94761084 on chromosome 1B (TRIDC1BG067780, TRIDC1BG067750, and TRIDC1BG067760) encode product with sequence homology to Arabidopsis BTB/POZ and MATH domain-containing protein 3 (BPM3). BPMs act as substrate-specific adapters for E3 ubiquitin-protein ligase complex (Chen et al., 2013), interacting with the negative regulator of flowering, MYB56, at the promoter of the floral activator, FLOWERING LOCUS T (Chen et al., 2015).
The most interesting candidate gene for QTL associated with AX-95219400 SNP on 3A is TRIDC3AG046200, with similarity to Arabidopsis ULTRAPETALA1 (ULT1), a trithorax group factor regulating transcriptional activation and counteracting EMF1 repressor function (Carles et al., 2005; Carles and Fletcher, 2009; Pu et al., 2013).
One of the candidate genes for AX-94918249 on chromosome 5A is TRIDC5AG000640, similar to Oryza sativa EARLY FLOWERING1 (EL1). It codes for CASEIN KINASE 1-LIKE protein HD16 involved in the regulation of flowering time through gibberellin signaling and photoperiod pathway under LD conditions (Hori et al., 2013; Kwon et al., 2015). AX-94918249 belongs to the QTL region QHd.cerz-5AS.1 associated with heading time, previously reported in T. turgidum L. ssp. durum (Roncallo et al., 2017).
An interesting candidate gene for another heading time-associated marker (AX-94609685) located on chromosome 5A is TRIDC5AG070010, similar to NAC domain-containing protein 37. NAC-domain protein LONG VEGETATIVE PHASE 1 (LOV1) was found to integrate and regulate flowering time and cold response in Arabidopsis through repression of CO under LD (Yoo et al., 2007). AX-94609685 is included in the QTL region QHd.cerz-5AL.3 associated with heading time in T. turgidum L. ssp. durum (Roncallo et al., 2017).
A candidate gene TRIDC6BG043170 on chromosome 6B (AX-94853271) encodes a protein similar to Cold-responsive protein kinase 1 that phosphorylates proteins and triggers their translocations from the cytosol to the nucleus in response to cold stress (Liu et al., 2017). Studies in wheat reported that cold-induced phosphorylation of VER2 is necessary for the VER2 transport from cytoplasm to nuclei, where it interacts with vernalization repressor GRP2 and releases the VRN1 pre-mRNA (Xing et al., 2009; Xiao et al., 2014; Kippes et al., 2018).
A possible candidate gene for AX-95172810 on chromosome 7B might be TRIDC7BG000850, similar to Arabidopsis B-box zinc finger protein 22. An example of a well-known Arabidopsis B-box zinc finger protein is CONSTANS (CO), promoting flowering under LD conditions (Putterill et al., 1995). One of the candidate genes is also Glutathione S-transferase T1 (GSTT1, TRIDC7BG000060), which homologs were identified as candidates for QTL related on heading date in the 7B chromosome arm substitution line of WE chromosome 7B in the genetic background of hexaploid Chinese Spring (Lu et al., 2022). It is well known that the GST is linked with diverse abiotic stresses. For instance, eighty-four GST genes were identified in barley and associated with an important role in drought tolerance (Rezaei et al., 2013). Even more (330) GST genes were identified in hexaploid wheat, and expression profiling of 14 selected genes indicated GST could respond to abiotic stress (Wang et al., 2019). In addition, four genes were upregulated under cold (Wang et al., 2019). Later, the number of GST genes identified in T. aestivum increased to 346 (Hao et al., 2021). GSTs were also found to be upregulated in response to cold in pepper (Capsicum annuum L.) (Islam et al., 2019) and pumpkins (Cucurbita maxima) (Abdul Kayum et al., 2018).
AX-95632383 located on chromosome 7B is included in the heading time-associated QTL QHd.cerz-7BL.3 from T. turgidum L. var. durum (Roncallo et al., 2017). We detected 15 candidate genes, but none have been reported to play a role in flowering.
4.2 VRN1 sequence variability
Of the four known vernalization response genes, the VRN1 gene is considered the most important one (Yan et al., 2003). Sequence analysis of the VRN1 gene in bread wheat revealed only minor sequence variation (Strejčková et al., 2021; Makhoul et al., 2022). This may reflect the limited gene pool of progenitors, which contributed to the original population(s) of bread wheat. To discover new alleles for potential use in the breeding of durum and bread wheat adapted to climate change, we sequenced the full-length VRN1 genes of wild emmer wheat genotypes collected in different areas of Fertile Crescent. Former studies investigating the VRN1 variability in wild emmer wheat relied on genotyping, restriction assays, and sequencing of short PCR products of the promoter or the first intron of VRN1 (Yan et al., 2004; Golovnina et al., 2010; Chhuneja et al., 2015; Shcherban et al., 2015; Konopatskaia et al., 2016).
Overall, high sequence variability was found in the panel of 95 wild emmer wheat genotypes. Interestingly, mutations were found in both non-coding and coding parts of the VRN1 gene. In total, we found 15 different SNPs in exons of VRN-A1 and seven SNPs, and one insertion in exons of VRN-B1. In VRN-A1, seven out of the 15 SNPs were non-synonymous, resulting in the amino acid sequence change. On the contrary, seven of the eight VRN-B1 exonic mutations changed the amino acid sequence. Gln88/His88 change in the K-box of VRN-A1 protein seems to be associated with later flowering. It was found in four Turkish winter genotypes (147-151 and 159-163 days to heading when scored in the field experiment in Turkey and Italy, respectively) with no other SNPs detected either in VRN-A1 or VRN-B1 alleles. Synonymous VRN-A1 exon2 SNP C8757T was identified only in three early winter genotypes carrying the VRN-A1b allele (134-139 (TUR) and 150-154 (IT) days to heading). Thr208/Ala208 amino acid change of VRN-B1 was found only in 13 early winter and five spring genotypes.
VRN1 alleles respond differently to environmental stimuli and have adaptive value to specific environments (Stelmakh, 1998). The observed sequence mutations within the VRN-A1 and VRN-B1 alleles formed eight and ten groups, respectively. Group 4 (VRN-A1 allele) was predominantly represented by WEW from Turkey, although several Turkey’s genotypes were found in other groups as well. Similarly, group 1 (VRN-A1 allele) mainly included the genotypes from Syria and Israel (Figure 6, Supplementary Table 19). The geographic distribution of VRN-B1 groups also showed that the vast majority of Turkey’s genotypes fell into the same group (Figure 7, Supplementary Table 20). These results suggest that genotypes clustering in the same group may share a pedigree and possibly a common ancestor. Although the frequencies of genotypes with different VRN1 alleles in different geographical regions suggest a possible adaptive role, additional studies using larger sample size will be necessary to quantify better the effect of the VRN1 alleles on the adaptation to different environments.
4.2.1 Partially characterized VRN1 alleles
Due to the limited sequencing technologies in the past, several VRN1 alleles are characterized only by a short partial promoter or intronic sequence. As shown before (Strejčková et al., 2021), the known allele VRN-A1b characterized by the 20-bp deletion in the promoter region (Yan et al., 2004) also carries a 177-bp insertion in the first intron. In our study, we found the same 177-bp insertion in the Vrn-A1d allele of PI 428054 (GenBank OP831152) and Tabigha 15 (GenBank OP831151), characterized so far only by 32-bp and 20-bp deletions in the promoter (Yan et al., 2004). Konopatskaia et al. (2016) proposed two possible mechanisms of Vrn-A1d origin: (1) from an extension of deletion in the VRN-A1b allele or (2) through the formation of two deletions in the vrn-A1 allele. Our data support the first hypothesis because both Vrn-A1d and VRN-A1b alleles share the 177-bp insertion in the intron 1.
The PI 560872 VRN-A1 allele shows high similarity with spring Vrn-A1f and Vrn-A1f-like alleles (Golovnina et al., 2010; Ivaničová et al., 2016) but lacks any indels in the intron 1, as described for Vrn-A1f-like. Unfortunately, the full-length sequence of Vrn-A1f is not available. Despite described mutations in the VRN-A1 promoter, the growth type for PI 560872 is determined as winter according to the GRIN-Global database (Version: 2.0.5.0), further supported by the heading time experiments. One can hypothesize that not the promoter variation but the intron 1 mutations are responsible for the spring phenotype in the Vrn-A1f and Vrn-A1f-like alleles. Civáň et al. (2013) and Badaeva et al. (2022) classified PI 560872 as T. timopheevii ssp. armeniacum.
4.2.2 Premature stop codon
The keratin-like (K-box) domain for VRN1 protein dimerization is postulated to form three amphipathic α-helices K1, K2, and K3 (Ma et al., 1991; Yang et al., 2003). Our study found a VRN-B1 protein with premature stop codon causing partial loss of K3 in the K-domain and complete loss of the C-terminal domain. Despite the mutation, PI 466991 was able to flower on average in 142 and 160 days when scored in the field experiments in Turkey and Italy, respectively. When grown under controlled conditions without vernalization treatment, only one out of seven plants survived and flowered in 199 days (data not shown). We can speculate that a functional VRN-A1 copy is sufficient for the transition into the reproductive stage. Moreover, the Δvrn1-null mutants demonstrated that complete VRN1 is not essential for flowering (Chen and Dubcovsky, 2012). Premature termination codon (PTC) can affect protein biosynthesis, gene expression, or mRNA stability (Gao et al., 2021; Liu et al., 2022). Transcripts of the mutant gene can be processed by nonsense-mediated mRNA decay, a mechanism for detecting and rapidly eliminating PTC-containing mRNAs (Maquat, 1995; Frischmeyer and Dietz, 1999; Hentze and Kulozik, 1999). Interestingly, the VRN-B1 expression level of other winter genotypes was significantly higher after four weeks of vernalization than the VRN-B1 expression level in PI 466991 (Figure 5). However, it is impossible to separate the effect of the PTC on the VRN-B1 expression from the mRNA stability.
4.2.3 Expression of mutant VRN1 alleles
The promoter region of the VRN1 gene contains multiple regulatory sites, and it is sufficient to induce transcription (Yan et al., 2004; Alonso-Peral et al., 2011). However, additional regulatory sites were found outside the promoter region (Fu et al., 2005). Our study identified several VRN1 amino acid substitutions in early and late flowering genotypes.
The gene expression analysis showed various effects of discovered mutations on transcript levels. Some mutations did not change the VRN-A1 expression, for instance, VRN-A1 K-box Gln88/His88 substitution in PI 538646 vrn-A1. Its expression was comparable to the vrn-A1b allele in the late flowering genotypes.
The PI 466935 VRN-A1b allele containing synonymous C8757T substitution in exon 2 showed the highest expression level. This mutation was found only in early flowering winter genotypes. The expression level of the vrn-B1 allele containing a 6-bp deletion in intron 7 was comparable to PI 466935.
Thr208/Ala208 substitution in the C-terminus of VRN-B1 of PI 471057 is associated with earlier winter and spring genotypes. Its expression was very high, increasing rapidly from two to four weeks of vernalization. It seems it also affects the expression of intact vrn-A1.
According to the currently accepted version of the ‘original’ vernalization model, activated VRN1 represses the flowering repressor VRN2 and upregulates the flowering activator FT, which further promotes the VRN1 expression (Chen and Dubcovsky, 2012; Debernardi et al., 2022). Tanaka et al. (2018) hypothesize that VRN1 binds the FT promoter directly and suggest that the late flowering phenotype of wheat with Ala180/Val180 substitution in VRN-A1 (Díaz et al., 2012; Li et al., 2013) might be caused by lower binding affinity of the changed protein to the FT promoter. This finding could explain the above-discussed changed expression of VRN1 alleles with exonic mutations or even altered expression of the intact homoeoallele. Based on the analysis of the VRN1 K-box, it is hypothesized that VRN1 acts as a tetramer (Itoh et al., 2021). Amino acid changes in the K-box and C-terminus responsible for the higher-order formations might hypothetically affect the tetramer formation and the binding affinity of the final VRN1 complex.
4.2.4 G4 motif deletions and Au SINE elements
G-quadruplex (G4) secondary structures can participate in the regulation of many cellular processes. Several studies in animals observed the direct role of G4 motifs in the regulation of replication, transcription, and mi-RNA expression (Huppert and Balasubramanian, 2007; Maizels and Gray, 2013; Valton et al., 2014; Lago et al., 2021; Spiegel et al., 2021; Zhu et al., 2021). In promoters of plants, strong co-occurrence of G4 motifs with transcription factors binding sites such as Telobox, MYB, and E2F motif was detected (Garg et al., 2016). Evidence of G4 motif significance in regulating gene expression in plants is emerging (Yang et al., 2020; Volná et al., 2021; Feng et al., 2022). Almost one million different G4 motifs were identified in bread wheat, enriched at the transcription start sites (TSS), first coding domain sequence (CDS), and the start codon (Cagirici and Sen, 2020). Cagirici and Sen (2020) also suggested a potential role of G4 in regulating VRN1 genes. The VRN-B1 promoter of two of the latest flowering winter genotypes (PI 560872 and PI 656872) carries the deletion of G4 in the VRN-B1 promoter. PI 560872 exhibits extremely late flowering when grown without vernalization in controlled conditions (43 days later than non-vernalized TDC, data not shown). Interestingly, it also possesses deletions in the VRN-A1 promoter known from spring alleles Vrn-A1f and Vrn-A1f-like, which have no early flowering effect in the PI 560872 genotype. Besides the deletion of the G4 motif in PI 560872 and PI 656872, there are also two complete Au SINE elements deletions in the intron 1 of VRN-B1, which could have a potentially negative effect on the flowering time since they can also be found in another late flowering genotype, PI 538659. Au SINE elements are non-long terminal repeat retrotransposons, which may affect the gene structure and function as they are able to create allelic variation (Keidar et al., 2018).
In conclusion, due to climate change and the growing human population, there will be a need to breed new cultivars that are more resilient to abiotic stress with higher yields. The wild emmer wheat genotypes provide additional variability for the flowering time response (Leigh et al., 2022). Here, we deliver diverse full-length sequences of the critical player of vernalization, the VRN1 gene. Our comprehensive sequence and expression analysis of the VRN1 alleles in the set of wild genotypes from Fertile Crescent provides an available reservoir of allelic diversity that could be introduced into breeding programs to expand the elite wheat gene pool. Moreover, the genome-wide association study (GWAS) results indicate other potential candidates for the fine-tuning of the flowering time.
Data availability statement
The datasets presented in this study can be found in online repositories. The names of the repository/repositories and accession number(s) can be found in the article/Supplementary Material.
Author contributions
JŠ and HÖ designed the study. BS conducted laboratory experiments. RČ performed GWAS analysis. BS, ZM, and RČ analyzed the data. ZM carried out a statistical analysis. BS, JŠ, ZM, and RČ wrote the manuscript. EM and HÖ developed, genotyped and characterised the wild emmer wheat collection, EÇ and AMM, phenotyped the collection in field experiments. JB analyzed the geographical distribution of VRN1 alleles. JŠ supervised the results. All authors contributed to the article and approved the submitted version.
Funding
This research was funded by Czech Science Foundation, grant number 22-00204S, by the Italian MUR (Ministero dell’Università e della Ricerca), grant CerealMed “Enhancing diversity in Mediterranean cereal farming systems”, in the frame of PRIMA Section 2—Multi-topic 2019, and by Cukurova University Scientific Research Coord., grant number FYL-2019-11954.
Acknowledgments
We are grateful for the excellent technical assistance of Tereza Vojtková and the sequencing service by Kateřina Holušová and Helena Tvardíková from the Czech Academy of Sciences, Institute of Experimental Botany. Computational resources were supplied by the project “e-Infrastruktura CZ” (e-INFRA LM2018140) provided within the program Projects of Large Research, Development and Innovations Infrastructures.
Conflict of interest
The authors declare that the research was conducted in the absence of any commercial or financial relationships that could be construed as a potential conflict of interest.
Publisher’s note
All claims expressed in this article are solely those of the authors and do not necessarily represent those of their affiliated organizations, or those of the publisher, the editors and the reviewers. Any product that may be evaluated in this article, or claim that may be made by its manufacturer, is not guaranteed or endorsed by the publisher.
Supplementary material
The Supplementary Material for this article can be found online at: https://www.frontiersin.org/articles/10.3389/fpls.2022.1106164/full#supplementary-material
References
Abdul Kayum, M., Nath, U., Park, J.-I., Biswas, M., Choi, E., Song, J.-Y., et al. (2018). Genome-wide identification, characterization, and expression profiling of glutathione s-transferase (GST) family in pumpkin reveals likely role in cold-stress tolerance. Genes (Basel). 9, 84. doi: 10.3390/genes9020084
Alonso-Peral, M. M., Oliver, S. N., Casao, M. C., Greenup, A. A., Trevaskis, B. (2011). The promoter of the cereal VERNALIZATION1 gene is sufficient for transcriptional induction by prolonged cold. PloS One 6, e29456. doi: 10.1371/journal.pone.0029456
Badaeva, E. D., Konovalov, F. A., Knüpffer, H., Fricano, A., Ruban, A. S., Kehel, Z., et al. (2022). Genetic diversity, distribution and domestication history of the neglected GGAtAt genepool of wheat. Theor. Appl. Genet. 135, 755–776. doi: 10.1007/s00122-021-03912-0
Balfourier, F., Bouchet, S., Robert, S., DeOliveira, R., Rimbert, H., Kitt, J., et al. (2019). Worldwide phylogeography and history of wheat genetic diversity. Sci. Adv. 5, eaav0536. doi: 10.1126/sciadv.aav0536
Balla, M. Y., Gorafi, Y. S. A., Kamal, N. M., Abdalla, M. G. A., Tahir, I. S. A., Tsujimoto, H. (2022a). Exploiting wild emmer wheat diversity to improve wheat a and b genomes in breeding for heat stress adaptation. Front. Plant Sci. 13. doi: 10.3389/fpls.2022.895742
Balla, M. Y., Gorafi, Y. S. A., Kamal, N. M., Abdalla, M. G. A., Tahir, I. S. A., Tsujimoto, H. (2022b). Harnessing the diversity of wild emmer wheat for genetic improvement of durum wheat. Theor. Appl. Genet. 135, 1671–1684. doi: 10.1007/s00122-022-04062-7
Bankevich, A., Nurk, S., Antipov, D., Gurevich, A. A., Dvorkin, M., Kulikov, A. S., et al. (2012). SPAdes: A new genome assembly algorithm and its applications to single-cell sequencing. J. Comput. Biol. 19, 455–477. doi: 10.1089/cmb.2012.0021
Beales, J., Turner, A., Griffiths, S., Snape, J. W., Laurie, D. A. (2007). A pseudo-response regulator is misexpressed in the photoperiod insensitive Ppd-D1a mutant of wheat (Triticum aestivum l.). Theor. Appl. Genet. 115, 721–733. doi: 10.1007/s00122-007-0603-4
Becker, A., Theißen, G. (2003). The major clades of MADS-box genes and their role in the development and evolution of flowering plants. Mol. Phylogenet. Evol. 29, 464–489. doi: 10.1016/S1055-7903(03)00207-0
Bolger, A. M., Lohse, M., Usadel, B. (2014). Trimmomatic: A flexible trimmer for illumina sequence data. Bioinformatics 30, 2114–2120. doi: 10.1093/bioinformatics/btu170
Cagirici, H. B., Sen, T. Z. (2020). Genome-wide discovery of G-quadruplexes in wheat: Distribution and putative functional roles. G3 Genes Genomes Genet. 10, 2021–2032. doi: 10.1534/g3.120.401288
Carles, C. C., Choffnes-Inada, D., Reville, K., Lertpiriyapong, K., Fletcher, J. C. (2005). ULTRAPETALA1 encodes a SAND domain putative transcriptional regulator that controls shoot and floral meristem activity in Arabidopsis. Development 132, 897–911. doi: 10.1242/dev.01642
Carles, C. C., Fletcher, J. C. (2009). The SAND domain protein ULTRAPETALA1 acts as a trithorax group factor to regulate cell fate in plants. Genes Dev. 23, 2723–2728. doi: 10.1101/gad.1812609
Chee, P. W., Elias, E. M., Anderson, J. A., Kianian, S. F. (2001). Evaluation of a high grain protein QTL from Triticum turgidum l. var. dicoccoides in an adapted durum wheat background. Crop Sci. 41, 295–301. doi: 10.2135/cropsci2001.412295x
Chen, L., Bernhardt, A., Lee, J., Hellmann, H. (2015). Identification of Arabidopsis MYB56 as a novel substrate for CRL3BPM E3 ligases. Mol. Plant 8, 242–250. doi: 10.1016/j.molp.2014.10.004
Chen, A., Dubcovsky, J. (2012). Wheat TILLING mutants show that the vernalization gene VRN1 down-regulates the flowering repressor VRN2 in leaves but is not essential for flowering. PloS Genet. 8, e1003134. doi: 10.1371/journal.pgen.1003134
Chen, L., Lee, J. H., Weber, H., Tohge, T., Witt, S., Roje, S., et al. (2013). Arabidopsis BPM proteins function as substrate adaptors to a CULLIN3-based E3 ligase to affect fatty acid metabolism in plants. Plant Cell 25, 2253–2264. doi: 10.1105/tpc.112.107292
Chhuneja, P., Arora, J. K., Kaur, P., Kaur, S., Singh, K. (2015). Characterization of wild emmer wheat Triticum dicoccoides germplasm for vernalization alleles. J. Plant Biochem. Biotechnol. 24, 249–253. doi: 10.1007/s13562-014-0281-7
Civáň, P., Ivaničová, Z., Brown, T. A. (2013). Reticulated origin of domesticated emmer wheat supports a dynamic model for the emergence of agriculture in the fertile crescent. PloS One 8, e81955. doi: 10.1371/journal.pone.0081955
Debernardi, J. M., Woods, D. P., Li, K., Li, C., Dubcovsky, J. (2022). MiR172-APETALA2-like genes integrate vernalization and plant age to control flowering time in wheat. PloS Genet. 18, e1010157. doi: 10.1371/journal.pgen.1010157
Díaz, A., Zikhali, M., Turner, A. S., Isaac, P., Laurie, D. A. (2012). Copy number variation affecting the photoperiod-B1 and vernalization-A1 genes is associated with altered flowering time in wheat (Triticum aestivum). PloS One 7, e33234. doi: 10.1371/journal.pone.0033234
Dubcovsky, J., Dvorak, J. (2007). Genome plasticity a key factor in the success of polyploid wheat under domestication. Science 316, 1862–1866. doi: 10.1126/science.1143986
Egea-Cortines, M., Saedler, H., Sommer, H. (1999). Ternary complex formation between the MADS-box proteins SQUAMOSA, DEFICIENS and GLOBOSA is involved in the control of floral architecture in Antirrhinum majus. EMBO J. 18, 5370–5379. doi: 10.1093/emboj/18.19.5370
Fadida-Myers, A., Fuerst, D., Tzuberi, A., Yadav, S., Nashef, K., Roychowdhury, R., et al. (2022). Emmer wheat eco-geographic and genomic congruence shapes phenotypic performance under Mediterranean climate. Plants 11, 1460. doi: 10.3390/plants11111460
Feng, Y., Tao, S., Zhang, P., Sperti, F. R., Liu, G., Cheng, X., et al. (2022). Epigenomic features of DNA G-quadruplexes and their roles in regulating rice gene transcription. Plant Physiol. 188, 1632–1648. doi: 10.1093/plphys/kiab566
Foreign Agricultural Service/USDA (2022). World agricultural production. Circ. Ser., 1–43. doi: 10.32317/2221-1055.201907059
Frischmeyer, P. A., Dietz, H. C. (1999). Nonsense-mediated mRNA decay in health and disease. Hum. Mol. Genet. 8, 1893–1900. doi: 10.1093/hmg/8.10.1893
Fu, D., Szucs, P., Yan, L., Helguera, M., Skinner, J. S., Von Zitzewitz, J., et al. (2005). Large Deletions within the first intron in VRN-1 are associated with spring growth habit in barley and wheat. Mol. Genet. Genomics 273, 54–65. doi: 10.1007/s00438-004-1095-4
Gao, Y., An, K., Guo, W., Chen, Y., Zhang, R., Zhang, X., et al. (2021). The endosperm-specific transcription factor TaNAC019 regulates glutenin and starch accumulation and its elite allele improves wheat grain quality. Plant Cell 33, 603–622. doi: 10.1093/plcell/koaa040
Garg, R., Aggarwal, J., Thakkar, B. (2016). Genome-wide discovery of G-quadruplex forming sequences and their functional relevance in plants. Sci. Rep. 6, 31–35. doi: 10.1038/srep28211
Golovnina, K. A., Kondratenko, E. Y., Blinov, A. G., Goncharov, N. P. (2010). Molecular characterization of vernalization loci VRN1 in wild and cultivated wheats. BMC Plant Biol. 10, 168. doi: 10.1186/1471-2229-10-168
Hao, Y., Xu, S., Lyu, Z., Wang, H., Kong, L., Sun, S. (2021). Comparative analysis of the glutathione s-transferase gene family of four Triticeae species and transcriptome analysis of GST genes in common wheat responding to salt stress. Int. J. Genomics 2021, 6289174. doi: 10.1155/2021/6289174
Harlan, J. R., Wet, J. M. J. (1971). Toward a rational classification of cultivated plants. Taxon 20, 509–517. doi: 10.2307/1218252
Hentze, M. W., Kulozik, A. E. (1999). A perfect message: RNA surveillance and nonsense-mediated decay. Cell 96, 307–310. doi: 10.1016/S0092-8674(00)80542-5
Hori, K., Ogiso-Tanaka, E., Matsubara, K., Yamanouchi, U., Ebana, K., Yano, M. (2013). Hd16, a gene for casein kinase I, is involved in the control of rice flowering time by modulating the day-length response. Plant J. 76, 36–46. doi: 10.1111/tpj.12268
Huang, M., Liu, X., Zhou, Y., Summers, R. M., Zhang, Z. (2019). BLINK: a package for the next level of genome-wide association studies with both individuals and markers in the millions. Gigascience 8, 1–12. doi: 10.1093/gigascience/giy154
Huppert, J. L., Balasubramanian, S. (2007). G-Quadruplexes in promoters throughout the human genome. Nucleic Acids Res. 35, 406–413. doi: 10.1093/nar/gkl1057
Islam, S., Sajib, S., Jui, Z. S., Arabia, S., Islam, T., Ghosh, A. (2019). Genome-wide identification of glutathione s-transferase gene family in pepper, its classification, and expression profiling under different anatomical and environmental conditions. Sci. Rep. 9, 9101. doi: 10.1038/s41598-019-45320-x
Itoh, T., Kamiya, A., Kimura, M., Murai, K. (2021). Heterogeneous expression and purification of the wheat VRN1 K-box domain suggest the formation of a tetramer of the VRN1 protein. Am. J. Plant Sci. 12, 1002–1010. doi: 10.4236/ajps.2021.127068
Ivaničová, Z., Jakobson, I., Reis, D., Šafář, J., Milec, Z., Abrouk, M., et al. (2016). Characterization of new allele influencing flowering time in bread wheat introgressed from Triticum militinae. N. Biotechnol. 33, 718–727. doi: 10.1016/j.nbt.2016.01.008
Ivaničová, Z., Valárik, M., Pánková, K., Trávníčková, M., Doležel, J., Šafář, J., et al. (2017). Heritable heading time variation in wheat lines with the same number of Ppd-B1 gene copies. PloS One 12, e0183745. doi: 10.1371/journal.pone.0183745
Jin, F. F., Wei, L. (2016). The expression patterns of three VRN genes in common wheat ( Triticum aestivum l.) in response to vernalization. Cereal Res. Commun. 44, 1–12. doi: 10.1556/0806.43.2015.041
Katoh, K., Misawa, K., Kuma, K., Miyata, T. (2002). MAFFT: a novel method for rapid multiple sequence alignment based on fast Fourier transform. Nucleic Acids Res. 30, 3059–3066. doi: 10.1093/nar/gkf436
Katoh, K., Standley, D. M. (2013). MAFFT multiple sequence alignment software version 7: Improvements in performance and usability. Mol. Biol. Evol. 30, 772–780. doi: 10.1093/molbev/mst010
Keidar, D., Doron, C., Kashkush, K. (2018). Genome-wide analysis of a recently active retrotransposon, au SINE, in wheat: content, distribution within subgenomes and chromosomes, and gene associations. Plant Cell Rep. 37, 193–208. doi: 10.1007/s00299-017-2213-1
Kippes, N., Guedira, M., Lin, L., Alvarez, M. A., Brown-Guedira, G. L., Dubcovsky, J. (2018). Single nucleotide polymorphisms in a regulatory site of VRN-A1 first intron are associated with differences in vernalization requirement in winter wheat. Mol. Genet. Genomics. 293, 1231–1243. doi: 10.1007/s00438-018-1455-0
Konopatskaia, I., Vavilova, V., Kondratenko, E. Y., Blinov, A., Goncharov, N. P (2016). VRN1 genes variability in tetraploid wheat species with a spring growth habit. BMC Plant Biol. 16, 244. doi: 10.1186/s12870-016-0924-z
Kwon, C.-T., Kim, S.-H., Kim, D., Paek, N.-C. (2015). The rice floral repressor Early flowering1 affects spikelet fertility by modulating gibberellin signaling. Rice 8, 23. doi: 10.1186/s12284-015-0058-1
Lago, S., Nadai, M., Cernilogar, F. M., Kazerani, M., Domíniguez Moreno, H., Schotta, G., et al. (2021). Promoter G-quadruplexes and transcription factors cooperate to shape the cell type-specific transcriptome. Nat. Commun. 12, 3885. doi: 10.1038/s41467-021-24198-2
Lai, X., Vega-Léon, R., Hugouvieux, V., Blanc-Mathieu, R., van der Wal, F., Lucas, J., et al. (2021). The intervening domain is required for DNA-binding and functional identity of plant MADS transcription factors. Nat. Commun. 12, 4760. doi: 10.1038/s41467-021-24978-w
Leigh, F. J., Wright, T. I. C., Horsnell, R. A., Dyer, S., Bentley, A. R. (2022). Progenitor species hold untapped diversity for potential climate-responsive traits for use in wheat breeding and crop improvement. Heredity (Edinb). 128, 291–303. doi: 10.1038/s41437-022-00527-z
Li, H. (2013). Aligning sequence reads, clone sequences and assembly contigs with BWA-MEM. arXiv: Genomics. doi: 10.48550/arXiv.1303.3997
Li, H., Handsaker, B., Wysoker, A., Fennell, T., Ruan, J., Homer, N., et al. (2009). The sequence Alignment/Map format and SAMtools. Bioinformatics 25, 2078–2079. doi: 10.1093/bioinformatics/btp352
Liu, G., Gao, Y., Wang, H., Wang, Y., Chen, J., Zhang, P., et al. (2022). Premature termination codon of 1Dy12 gene improves cookie quality in Ningmai9 wheat. Front. Plant Sci. 13. doi: 10.3389/fpls.2022.835164
Liu, X., Huang, M., Fan, B., Buckler, E. S., Zhang, Z. (2016). Iterative usage of fixed and random effect models for powerful and efficient genome-wide association studies. PloS Genet. 12, e1005767. doi: 10.1371/journal.pgen.1005767
Liu, Z., Jia, Y., Ding, Y., Shi, Y., Li, Z., Guo, Y., et al. (2017). Plasma membrane CRPK1-mediated phosphorylation of 14-3-3 proteins induces their nuclear import to fine-tune CBF signaling during cold response. Mol. Cell 66, 117–128.e5. doi: 10.1016/j.molcel.2017.02.016
Li, G., Yu, M., Fang, T., Cao, S., Carver, B. F., Yan, L. (2013). Vernalization requirement duration in winter wheat is controlled by TaVRN-A1 at the protein level. Plant J. 76, 742–753. doi: 10.1111/tpj.12326
Lu, F., Chen, M., Zhao, Y., Wu, S., Yasir, M., Zhang, H., et al. (2022). Genetic mapping and candidate gene prediction of a QTL related to early heading on wild emmer chromosome 7BS in the genetic background of common wheat. Agronomy 12, 1089. doi: 10.3390/agronomy12051089
Maccaferri, M., Harris, N. S., Twardziok, S. O., Pasam, R. K., Gundlach, H., Spannagl, M., et al. (2019). Durum wheat genome highlights past domestication signatures and future improvement targets. Nat. Genet. 51, 885–895. doi: 10.1038/s41588-019-0381-3
Maizels, N., Gray, L. T. (2013). The G4 genome. PloS Genet. 9, e1003468. doi: 10.1371/journal.pgen.1003468
Makhoul, M., Chawla, H. S., Wittkop, B., Stahl, A., Voss-Fels, K. P., Zetzsche, H., et al. (2022). Long-amplicon single-molecule sequencing reveals novel, trait-associated variants of VERNALIZATION1 homoeologs in hexaploid wheat. Front. Plant Sci. 13. doi: 10.3389/fpls.2022.942461
Maquat, L. E. (1995). When cells stop making sense: effects of nonsense codons on RNA metabolism in vertebrate cells. RNA 1, 453–465.
Ma, H., Yanofsky, M. F., Meyerowitz, E. M. (1991). AGL1-AGL6, an Arabidopsis gene family with similarity to floral homeotic and transcription factor genes. Genes Dev. 5, 484–495. doi: 10.1101/gad.5.3.484
Mayer, K. F. X., Rogers, J., Doležel, J., Pozniak, C., Eversole, K., Feuillet, C., et al. (2014). A chromosome-based draft sequence of the hexaploid bread wheat (Triticum aestivum) genome. Science 345, 1251788. doi: 10.1126/science.1251788
Merchuk-Ovnat, L., Barak, V., Fahima, T., Ordon, F., Lidzbarsky, G. A., Krugman, T., et al. (2016). Ancestral QTL alleles from wild emmer wheat improve drought resistance and productivity in modern wheat cultivars. Front. Plant Sci. 7. doi: 10.3389/fpls.2016.00452
Mulder, N. J., Apweiler, R., Attwood, T. K., Bairoch, A., Bateman, A., Binns, D., et al. (2007). New developments in the InterPro database. Nucleic Acids Res. 35, 224–228. doi: 10.1093/nar/gkl841
Muterko, A., Kalendar, R., Salina, E. (2016). Novel alleles of the VERNALIZATION1 genes in wheat are associated with modulation of DNA curvature and flexibility in the promoter region. BMC Plant Biol. 16, 65–81. doi: 10.1186/s12870-015-0691-2
Nevo, E., Fu, Y.-B., Pavlicek, T., Khalifa, S., Tavasi, M., Beiles, A. (2012). Evolution of wild cereals during 28 years of global warming in Israel. Proc. Natl. Acad. Sci. 109, 3412–3415. doi: 10.1073/pnas.1121411109
Nuttonson, M. Y. (1955). Wheat-Climate Relationships and the Use of Phenology in Ascertaining the Thermal and Photo-Thermal Requirements of Wheat. Washington, DC: American Institute of Crop Ecology, 388pp.
Peng, J. H., Fahima, T., Röder, M. S., Huang, Q. Y., Dahan, A., Li, Y. C., et al. (2000). High-density molecular map of chromosome region harboring stripe-rust resistance genes YrH52 and Yr15 derived from wild emmer wheat, triticum dicoccoides. Genetica 109, 199–210. doi: 10.1023/A:1017573726512
Peng, J., Ronin, Y., Fahima, T., Röder, M. S., Li, Y., Nevo, E., et al. (2003). Domestication quantitative trait loci in Triticum dicoccoides , the progenitor of wheat. Proc. Natl. Acad. Sci. 100, 2489–2494. doi: 10.1073/pnas.252763199
Piepho, H. P., Möhring, J., Melchinger, A. E., Büchse, A. (2008). BLUP for phenotypic selection in plant breeding and variety testing. Euphytica 161, 209–228. doi: 10.1007/s10681-007-9449-8
Pu, L., Liu, M., Kim, S. Y., Chen, L. F. O., Fletcher, J. C., Renee Sung, Z. (2013). EMBRYONIC FLOWER1 and ULTRAPETALA1 act antagonistically on arabidopsis development and stress response. Plant Physiol. 162, 812–830. doi: 10.1104/pp.112.213223
Putterill, J., Robson, F., Lee, K., Simon, R., Coupland, G. (1995). The CONSTANS gene of arabidopsis promotes flowering and encodes a protein showing similarities to zinc finger transcription factors. Cell 80, 847–857. doi: 10.1016/0092-8674(95)90288-0
Reader, S. M., Miller, T. E. (1991). The introduction into bread wheat of a major gene for resistance to powdery mildew from wild emmer wheat. Euphytica 53, 57–60. doi: 10.1007/BF00032033
Rezaei, M. K., Shobbar, Z. S., Shahbazi, M., Abedini, R., Zare, S. (2013). Glutathione s-transferase (GST) family in barley: Identification of members, enzyme activity, and gene expression pattern. J. Plant Physiol. 170, 1277–1284. doi: 10.1016/j.jplph.2013.04.005
Rice, P., Longden, L., Bleasby, A. (2000). EMBOSS: The European molecular biology open software suite. Trends Genet. 16, 276–277. doi: 10.1016/S0168-9525(00)02024-2
Robinson, J. T., Thorvaldsdóttir, H., Wenger, A. M., Zehir, A., Mesirov, J. P. (2017). Variant review with the integrative genomics viewer. Cancer Res. 77, e31–e34. doi: 10.1158/0008-5472.CAN-17-0337
Roncallo, P. F., Akkiraju, P. C., Cervigni, G. L., Echenique, V. C. (2017). QTL mapping and analysis of epistatic interactions for grain yield and yield-related traits in Triticum turgidum l. var. durum. Euphytica 213, 277. doi: 10.1007/s10681-017-2058-2
Rong, J. K., Millet, E., Manisterski, J., Feldman, M. (2000). A new powdery mildew resistance gene: Introgression from wild emmer into common wheat and RFLP-based mapping. Euphytica 115, 121–126. doi: 10.1023/A:1003950431049
Royo, C., Soriano, J. M., Alvaro, F. (2017). “Wheat: A crop in the bottom of the Mediterranean diet pyramid,” in Mediterranean identities - environment, society. Culture (InTech), 381–399. doi: 10.5772/intechopen.69184
Saedler, H., Becker, A., Winter, K. U., Kirchner, C., Theißen, G. (2001). MADS-box genes are involved in floral development and evolution. Acta Biochim. Pol. 48, 351–358. doi: 10.18388/abp.2001_3920
Saitou, N., Nei, M. (1987). The neighbor-joining method: a new method for reconstructing phylogenetic trees. Mol. Biol. Evol. 4, 406–425. doi: 10.1093/oxfordjournals.molbev.a040454
Shcherban, A. B., Schichkina, A. A., Salina, E. A. (2016). The occurrence of spring forms in tetraploid Timopheevi wheat is associated with variation in the first intron of the VRN-A1 gene. BMC Plant Biol. 16, 236. doi: 10.1186/s12870-016-0925-y
Shcherban, A. B., Strygina, K. V., Salina, E. A. (2015). VRN-1 gene- associated prerequisites of spring growth habit in wild tetraploid wheat T. dicoccoides and the diploid a genome species. BMC Plant Biol. 15, 94. doi: 10.1186/s12870-015-0473-x
Spiegel, J., Cuesta, S. M., Adhikari, S., Hänsel-Hertsch, R., Tannahill, D., Balasubramanian, S. (2021). G-Quadruplexes are transcription factor binding hubs in human chromatin. Genome Biol. 22, 117. doi: 10.1186/s13059-021-02324-z
Stelmakh, A. F. (1998). Genetic systems regulating flowering response in wheat. Euphytica 100, 359–369. doi: 10.1023/a:1018374116006
Strejčková, B., Milec, Z., Holušová, K., Cápal, P., Vojtková, T., Čegan, R., et al. (2021). In-depth sequence analysis of bread wheat VRN1 genes. Int. J. Mol. Sci. 22, 12284. doi: 10.3390/ijms222212284
Tamura, K., Nei, M. (1993). Estimation of the number of nucleotide substitutions in the control region of mitochondrial DNA in humans and chimpanzees. Mol. Biol. Evol. 10, 512–526. doi: 10.1093/oxfordjournals.molbev.a040023
Tanaka, C., Itoh, T., Iwasaki, Y., Mizuno, N., Nasuda, S., Murai, K. (2018). Direct interaction between VRN1 protein and the promoter region of the wheat FT gene. Genes Genet. Syst. 93, 25–29. doi: 10.1266/ggs.17-00041
Trevaskis, B., Bagnall, D. J., Ellis, M. H., Peacock, W. J., Dennis, E. S. (2003). MADS box genes control vernalization-induced flowering in cereals. Proc. Natl. Acad. Sci. 100, 13099–13104. doi: 10.1073/pnas.1635053100
Valton, A. L., Hassan-Zadeh, V., Lema, I., Boggetto, N., Alberti, P., Saintomé, C., et al. (2014). G4 motifs affect origin positioning and efficiency in two vertebrate replicators. EMBO J. 33, 732–746. doi: 10.1002/embj.201387506
Volná, A., Bartas, M., Nezval, J., Špunda, V., Pečinka, P., Červeň, J. (2021). Searching for G-Quadruplex-Binding proteins in plants: New insight into possible G-quadruplex regulation. BioTech 10, 20. doi: 10.3390/biotech10040020
Wang, R., Ma, J., Zhang, Q., Wu, C., Zhao, H., Wu, Y., et al. (2019). Genome-wide identification and expression profiling of glutathione transferase gene family under multiple stresses and hormone treatments in wheat (Triticum aestivum l.). BMC Genomics 20, 986. doi: 10.1186/s12864-019-6374-x
Xiao, J., Xu, S., Li, C., Xu, Y., Xing, L., Niu, Y., et al. (2014). O-GlcNAc-mediated interaction between VER2 and TaGRP2 elicits TaVRN1 mRNA accumulation during vernalization in winter wheat. Nat. Commun. 5, 4572. doi: 10.1038/ncomms5572
Xing, L., Li, J., Xu, Y., Xu, Z., Chong, K. (2009). Phosphorylation modification of wheat lectin VER2 is associated with vernalization-induced O-GlcNAc signaling and intracellular motility. PloS One 4, e4854. doi: 10.1371/journal.pone.0004854
Yang, X., Cheema, J., Zhang, Y., Deng, H., Duncan, S., Umar, M. I., et al. (2020). RNA G-Quadruplex structures exist and function in vivo in plants. Genome Biol. 21, 226. doi: 10.1186/s13059-020-02142-9
Yang, Y., Fanning, L., Jack, T. (2003). The K domain mediates heterodimerization of the Arabidopsis floral organ identity proteins, APETALA3 and PISTILLATA. Plant J. 33, 47–59. doi: 10.1046/j.0960-7412.2003.01473.x
Yan, L., Helguera, M., Kato, K., Fukuyama, S., Sherman, J., Dubcovsky, J. (2004). Allelic variation at the VRN-1 promoter region in polyploid wheat. Theor. Appl. Genet. 109, 1677–1686. doi: 10.1007/s00122-004-1796-4
Yan, L., Loukoianov, A., Tranquilli, G., Helguera, M., Fahima, T., Dubcovsky, J. (2003). Positional cloning of the wheat vernalization gene VRN1. Proc. Natl. Acad. Sci. 100, 6263–6268. doi: 10.1073/pnas.0937399100
Yoo, S. Y., Kim, Y., Kim, S. Y., Lee, J. S., Ahn, J. H. (2007). Control of flowering time and cold response by a NAC-domain protein in Arabidopsis. PloS One 2, e642. doi: 10.1371/journal.pone.0000642
Keywords: VERNALIZATION1, next generation sequencing, heading time, GWAS, wild emmer wheat
Citation: Strejčková B, Mazzucotelli E, Čegan R, Milec Z, Brus J, Çakır E, Mastrangelo AM, Özkan H and Šafář J (2023) Wild emmer wheat, the progenitor of modern bread wheat, exhibits great diversity in the VERNALIZATION1 gene. Front. Plant Sci. 13:1106164. doi: 10.3389/fpls.2022.1106164
Received: 23 November 2022; Accepted: 16 December 2022;
Published: 06 January 2023.
Edited by:
Liang Wu, Zhejiang University, ChinaReviewed by:
Zujun Yang, University of Electronic Science and Technology of China, ChinaChanghui Sun, Sichuan Agricultural University, China
Copyright © 2023 Strejčková, Mazzucotelli, Čegan, Milec, Brus, Çakır, Mastrangelo, Özkan and Šafář. This is an open-access article distributed under the terms of the Creative Commons Attribution License (CC BY). The use, distribution or reproduction in other forums is permitted, provided the original author(s) and the copyright owner(s) are credited and that the original publication in this journal is cited, in accordance with accepted academic practice. No use, distribution or reproduction is permitted which does not comply with these terms.
*Correspondence: Jan Šafář, safar@ueb.cas.cz