- 1Advanced Plant Physiology Section, Department of Botany, Aligarh Muslim University, Aligarh, India
- 2Department of Integrated Forest and Landscape Protection, Faculty of Forestry, Technical University in Zvolen, Zvolen, Slovakia
- 3Global Change Research Institute, Czech Academy of Sciences, Brno, Czechia
The cultivation of lemongrass (Cymbopogon flexuosus) crop is dominated by its medicinal, food preservative, and cosmetic demands. The growing economy of the lemongrass market suggests the immense commercial potential of lemongrass and its essential oil. Nevertheless, the continuous increase of the saline regime threatens the growth and productivity of most of the plant life worldwide. In this regard, the present experiment explores the salt sensitiveness of the lemongrass crop against five different levels of salt stress. Metabolomic analyses suggest that lemongrass plants can effectively tolerate a salt concentration of up to 80 mM and retain most of their growth and productivity. However, extreme NaCl concentrations (≥160 mM) inflicted significant (α = 0.05) damage to the plant physiology and exhausted the lemongrass antioxidative defence system. Therefore, the highest NaCl concentration (240 mM) minimised plant height, chlorophyll fluorescence, and essential oil production by up to 50, 27, and 45%. The overall data along with the salt implications on photosynthetic machinery and ROS metabolism suggest that lemongrass can be considered a moderately sensitive crop to salt stress. The study, sensu lato, can be used in reclaiming moderately saline lands with lemongrass cultivation converting such lands from economic liability to economic asset.
Introduction
Lemongrass (Cymbopogon flexuosus) is a C4 perennial cash crop belonging to the Poaceae family and is commonly known as East Indian or Malabar grass. The major share of lemongrass cultivation is based on its essential oil production. The lemongrass essential oil (LEO) has enormous medicinal and commercial potential. The pharmacological benefits of LEO are derived from its antimicrobial, anticancer, insect-repellent, and antioxidant properties (Mukarram et al., 2021a,b). These bioactivities are further exploited in cosmetics and food packaging and safety. Lemongrass comprises a rich source of vitamins (A, C, E, folate, niacin, and riboflavin), protein, and mineral nutrients (Fe, Mn, Zn, Ca, N, P, K) making it an excellent nutritional source (Aftab et al., 2011; Gaba et al., 2020). The high cellulosic (cellulose, 39.5%; hemicellulose, 22.6%) structure of lemongrass along with lignin (28.5%) abundance make it a suitable candidate for the synthesis of vitamin A, β- and methyl-ionones, paper, pulp, silica, and composites (Haque et al., 2018). Few recent studies suggested that lemongrass can also be used to produce biofuel (Dhinesh et al., 2016; Sathiyamoorthi and Sankaranarayanan, 2016; Venkatesan et al., 2019). The perspective research into these lesser developed sectors can further augment the importance of lemongrass plants. The immense benefits of lemongrass and its essential oil are nudging crop scientists to develop new sustainable methods of enhancing lemongrass growth and productivity (Mukarram et al., 2021c,d).
On a global scale, the lemongrass is harvested over 16,000 ha of land which corresponds to an annual production of 1000 t of LEO (Haque et al., 2018). Mukarram et al. (2021b) reviewed the lemongrass economics and suggested a rapid growing pattern in India and globally as well. It was suggested that the estimated LEO market in 2019 was 247 million US$ which will be approximately doubled by 2027. Moreover, the Indian export of lemongrass was escalated with more than 1250% growth in the past two decades (2001–2020). Nevertheless, considering the soaring pattern, the demand for lemongrass and its essential oil is expected to be intensified in the upcoming years.
Massive population growth and urbanisation are discouraging agricultural lands and degrading the soil. Soil salinity is one of the leading threats to agricultural production worldwide. More than 800 million hectares are said to be salt-affected, which accounts for about 27 billion US$ global economic loss each year (Qadir et al., 2014). Soil is called saline when its electrical conductivity exceeds or equals 4 dS m–1 which is equivalent to 40 mM of NaCl (USSL, 2005). While primary salinity is naturally occurring, secondary salinity is largely contributed by anthropogenic activities including improper soil clearing and irrigational practices (Pitman and Läuchli, 2002; Munns, 2005). Most crops are glycophytes and cannot grow well in high salt concentrations. Thus, depending on the severity, salinity can regulate seed germination, cell expansion, stomatal conductance, photosynthesis, and other metabolic and developmental pathways (Shabala and Cuin, 2008; Machado and Serralheiro, 2017). In such crops, salinity can inflict moderate to acute harm depending on certain factors such as salt concentration and plant resilience (Munns and Tester, 2008). The modus operandi of salinity stress occurs in two phases: The first phase imposes osmotic stress on plants by restricting the water uptake that mimics drought-like situations (Munns, 2002). The osmotic phase distorts the ion homoeostasis and builds ion excess in the plant (ionic stress phase) in a salt-specific effect (van Zelm et al., 2020). These primary implications endorse a more comprehensive range of secondary effects induced by the saline regime such as oxidative stress and nutritional imbalance (Negrão et al., 2017). The ion-excess effect arises when salt concentration hits toxic levels inside the leaves. Therefore, the plants first try to minimise the salt uptake through roots and later separate the remaining at the cellular and tissue level to evade excessive salt accumulation in the cytoplasm of photosynthetic leaves (Munns, 2005; Flowers and Colmer, 2008). The osmotic stress and ionic stress support the overproduction of various reactive species that contributes to oxidative stress. Although reactive oxygen species (ROS) are produced as a by-product of many physiological processes including photosynthesis and respiration under the normal environment that can facilitate plant signalling, their overproduction during abiotic stress poses greater oxidative stress that damages proteins, lipids, and nucleic acids and limits crop growth and yield (Ashraf, 2009; Mukarram et al., 2021e). Similar salt-induced restrictions on plant development and productivity were observed in many crops of economic importance including rice, wheat, barley, and maize (Zeng and Shannon, 2000; Munns et al., 2006; Widodo et al., 2009; Zörb et al., 2019; Mukarram et al., 2021f).
Keeping in the mind the decreasing agricultural landholdings and the growing demand for lemongrass and its oil, we conducted the present experiment to assess the salt-sensitivity of the lemongrass crop. We hypothesised that the lemongrass, being a member of the grass family, would withstand the low (40 mM NaCl) to moderate (80 mM NaCl) salinity. The present experiment is an attempt to suggest to the wider audience the cultivation of lemongrass crops in lands deemed unsuitable for traditional cereal crops due to soil salinity. Furthermore, though lemongrass has a well-defined defence system comprising enzymatic and non-enzymatic antioxidants (Aziz et al., 2014) that might counter salinity-induced oxidative stress, it would be interesting to observe the potential of this defence system against severe salinity (≥160 mM).
Materials and Methods
Plant Material and Growth Conditions
The present study was performed at the net house of the Department of Botany, Aligarh Muslim University, Aligarh, India (27°52′ N, 78°51′ E, 187 m a.s.l.). The lemongrass [Cymbopogon flexuosus (Steud.) Wats var. Nima] was propagated from healthy slips brought from Central Institute for Medicinal and Aromatic Plants, Lucknow, India. The slips of the plants were sterilised with 0.2% HgCl2 and washed afterward repetitively. The plant slips were transferred to the 7 L capacity earthen pots (25 cm × 25 cm) mixed with sand, clay, and peat in a 7/2/1 ratio of their weights. Plants were irrigated with 250 mL of double-distilled water (DDW) daily. The average temperature and humidity were recorded as 17–25°C (±4°C), and 68% (±5%) during the experimental timeline. Soil evaluation at the Soil Testing Laboratory of Indian Agriculture Research Institute (IARI), New Delhi, India, described the soil physical texture as sandy loam while chemical variables were as follows: pH, 7.6; electrical conductivity, 0.52 dS m–1; N, 94.8 mg kg–1 of soil; P, 8.9 mg kg–1 of soil; K, 136.5 mg kg–1 of soil.
Plants were cultivated after 30 days from the final salt treatment for all the morpho-physiological assessments. The experimental setup was arranged in a complete random block design. Three lemongrass plants were grown in each pot, and each pot was considered as one replicate. Each treatment was replicated three times.
Induction of Salinity Stress
Five different salt regimes (0, 40, 80, 160, and 240 mM) were created for the present study (Table 1). A salt concentration of 40 mM NaCl was provided to the lemongrass plants on alternate days to induce these saline regimes. Thus, it took 1 day to attain required salt concentration for Treatment 2 (40 mM NaCl), 3 days for Treatment 3 (80 mM NaCl), 7 days for Treatment 4 (160 mM NaCl), and 11 days for Treatment 5 (240 mM NaCl). To maintain uniform salinity the above salt concentration was given in the form of an aqueous solution (40 mL). If the salt stress is added in one go, the plant might not grow at all and could take considerable time to recover, depending on the level of osmotic stress it caused. Therefore, salt treatments in the present study were added gradually to attain the required NaCl level and prevent the plant from having osmotic shock.
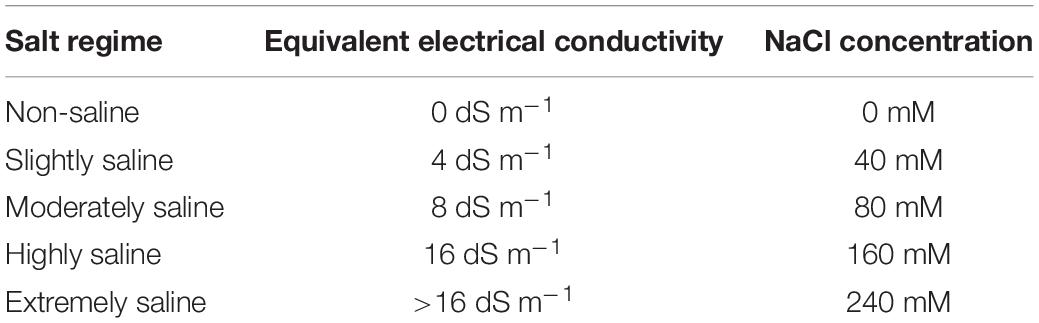
Table 1. Different salt regimes generated during the present experiment for assessing salt tolerance in lemongrass.
The salt conditions were devised based on the classification provided by the US Salinity Laboratory (Richard, 1954) to gauge the salinity tolerance level of lemongrass. Therefore, the present study consisted following salt regimes.
Figure 1 illustrates all the major and significant events in the experimental timeline, from the growing of the lemongrass plants to their harvesting.
Quantification of Photosynthetic Fitness
The chlorophyll content was determined from the completely expanded lemongrass leaves by using the Minolta chlorophyll metre (SPAD-502, Konica Minolta Sensing Inc., Osaka, Japan).
Chlorophyll fluorescence (Fv/Fm) was expressed as the quantum efficiency of open photosystem II (PSII) centres, which were dark-adapted for 30 min before measurement. Fv/Fm was determined in the daytime on the adaxial surface of the first fully developed leaf by a chlorophyll fluorometer, PAM-2000 (Walz, Effeltrich, Germany).
Gas Exchange
The gas exchange between the plant and the external environment was determined in terms of net photosynthetic rate (PN), stomatal conductance (gs), and transpiration rate (E). All the variables were quantified in the first expanded plant leaf through an Infra-red Gas Analyser (LI-COR 6200, Portable Photosynthesis System, NA, United States). The leaf area for the assessment was 6 cm2. The gas exchange was analysed at light-saturating intensity (PAR: 780–800 μmol m–2 s–1) with an air temperature of 25°C, relative humidity 65–85%, and 370 ± 5 μmol mol–1 atmospheric CO2 concentration.
Markers for Oxidative Damage Assessment
Thiobarbituric Acid Reactive Substances Content
The TBARS concentration was assessed to determine the lipid peroxidation in lemongrass leaves according to Cakmak and Horst (1991). Fresh lemongrass leaves (0.5 g) were ground in trichloroacetic acid (TCA, 5 ml, 0.1% w/v) followed by centrifugation (12,000 × g, 5 min). The supernatant (1 mL) along with tetrabutylammonium (4 mL, 0.5% w/v) was mixed with TCA (20% w/v) and incubated (90°C, 30 min) followed by ice bath treatment and a second round of centrifugation (10,000 × g, 5 min). The TBARS content was calculated as malondialdehyde equivalents by noting the absorbance of the mixture spectrophotometrically (Shimadzu UV-1700, Tokyo, Japan) at 532 nm followed by the subtraction of the absorbance recorded at 600 nm for further correction of non-specific turbidity.
Hydrogen Peroxide Content
The H2O2 content was calculated through a peroxidase-dependent essay as developed by Okuda et al. (1991) to estimate the oxidative imbalance in the salt-stressed lemongrass. The plant leaves were sampled and ground in perchloric acid. The subsequent solution was centrifuged and blended with peroxidase to commence the reaction. The optical density of the reaction mixture was noted for 3 min at 590 nm.
Lemongrass Defence System
Preparation of Enzyme Extract
The lemongrass leaves were cut and frozen with liquid nitrogen. The frozen leaves were crushed using a mortar and pestle into a powder which was mixed with an extraction solution (5 mL w/v) comprising potassium phosphate buffer (100 mM, pH 7.8), polyvinylpyrrolidone (1% w/v), and triton-X-100 (0.5% v/v). Afterward, the mixture was centrifuged (15,000 × g, 5 min, 4°C) and the supernatant was utilised to distinguish various antioxidants activities (Kuo et al., 1982).
Superoxide Dismutase Activity
The methods as developed by Beauchamp and Fridovich (1971) were followed to mark SOD (E.C. 1.15.1.1) activity. A reaction mixture (4 mL) of enzyme extract (0.1 mL) with riboflavin (1 mM), methionine (9.9 mM), triton-X-100 (0.02%), nitro blue tetrazolium (NBT, 55 mM), and EDTA (2 mM) was illuminated and sustained (30°C, 1 h). Later, the absorbance was noted at 560 nm and SOD activity was expressed as the amount needed to half inhibit the NBT reaction.
Catalase Activity
The CAT (E.C. 1.11.1.6) activity was assessed following a similar procedure as explained in our previous report (Mukarram et al., 2021d). A reaction mixture with enzyme extract (0.04 mL), potassium phosphate buffer (2.6 mL, 50 mM, pH 7), and H2O2 (0.4 mL, 15 mM) was prepared and centrifuged (12,500 × g, 20 min, 4°C). Later, CAT activity was calculated from H2O2 disappearance at 240 nm.
Peroxidase Activity
The activity of the POX (E.C. 1.11.1.7) enzyme was detected according to Kumar and Khan (1982). The enzymatic activity for POX was expressed as the conversion rate of pyrogallol to purpurogallin at 420 nm.
Proline Content
The osmolyte proline was quantified in lemongrass leaves using an earlier protocol (Bates et al., 1973). Fresh leaves (0.25 g) were chopped in sulfosalicylic acid (2.5 mL, 3%) and centrifuged (10,000 × g, 10 min), followed by water-bath boiling (100°C, 1 h) with a mixture of sulfosalicylic acid (2.5 mL), glacial acetic acid (1 mL), and acid ninhydrin solution (1 mL). The addition of toluene (3 mL) initiated chromophore generation whose absorbance was noted at 520 nm for proline estimation.
Determination of Growth Variables
The plant growth was assessed on three variables, i.e., plant height, dry weight, and leaf area. The dry weight was determined after drying the plant for 24 h in a hot-air oven at 80°C. The dried plants were then weighed using an electric balance.
Essential Oil Machinery
Geraniol Dehydrogenase Activity
Geraniol dehydrogenase (E.C. 1.1.1.183) is a key enzyme in essential oil biosynthesis in lemongrass plants. To address the impact of salinity on lemongrass oil biosynthesis machinery, GeDH activity was determined as described in our earlier experiment (Mukarram et al., 2021c). A reaction mixture (pH 7.5) was prepared by grounding lemongrass leaf tissue in tricine-NaOH, Polyclar AT, glycerol, and β-mercaptoethanol, thiourea. The geraniol dependent-NADP+ conversion was used as a marker for GeDH activity and was expressed in n katal mg–1 protein.
Essential Oil Productivity
The essential oil production was determined by the gravimetrical method developed by Guenther (1972). Fresh lemongrass leaves (100 g) were cut into tiny pieces and transferred to a flask attached to the Clevenger’s apparatus (Borosil, India). The cut leaves were boiled in DDW in the flask for 3 h using a heating mantle. The vapour formed contained the essential oil mixed with DDW. This vapour was cooled down using a condenser and captured into the receiver.
Statistical Analyses and Graphical Presentations
The normal distribution of the data was first tested for each treatment by the Shapiro–Wilk test. The homogeneity of variance among treatments was tested with Bartlett’s test. One-way analysis of variance (ANOVA) was used to test the salt effect on lemongrass growth, development, and productivity. Duncan’s multiple range post hoc test was used to determine the significance of differences among the treatments. Correlation analysis was used to analyse relationships between all parameters observed for control and four stress treatments. Principal component analysis was applied to measured parameters to explore the overall relationship between them and examine the positioning of the treatments within this system. All statistical analyses were conducted at the replicate level and α = 0.05 in SPSS-25.0 for Windows (SPSS, Inc., Chicago, IL, United States). Figure 2 was created with BioRender.com.
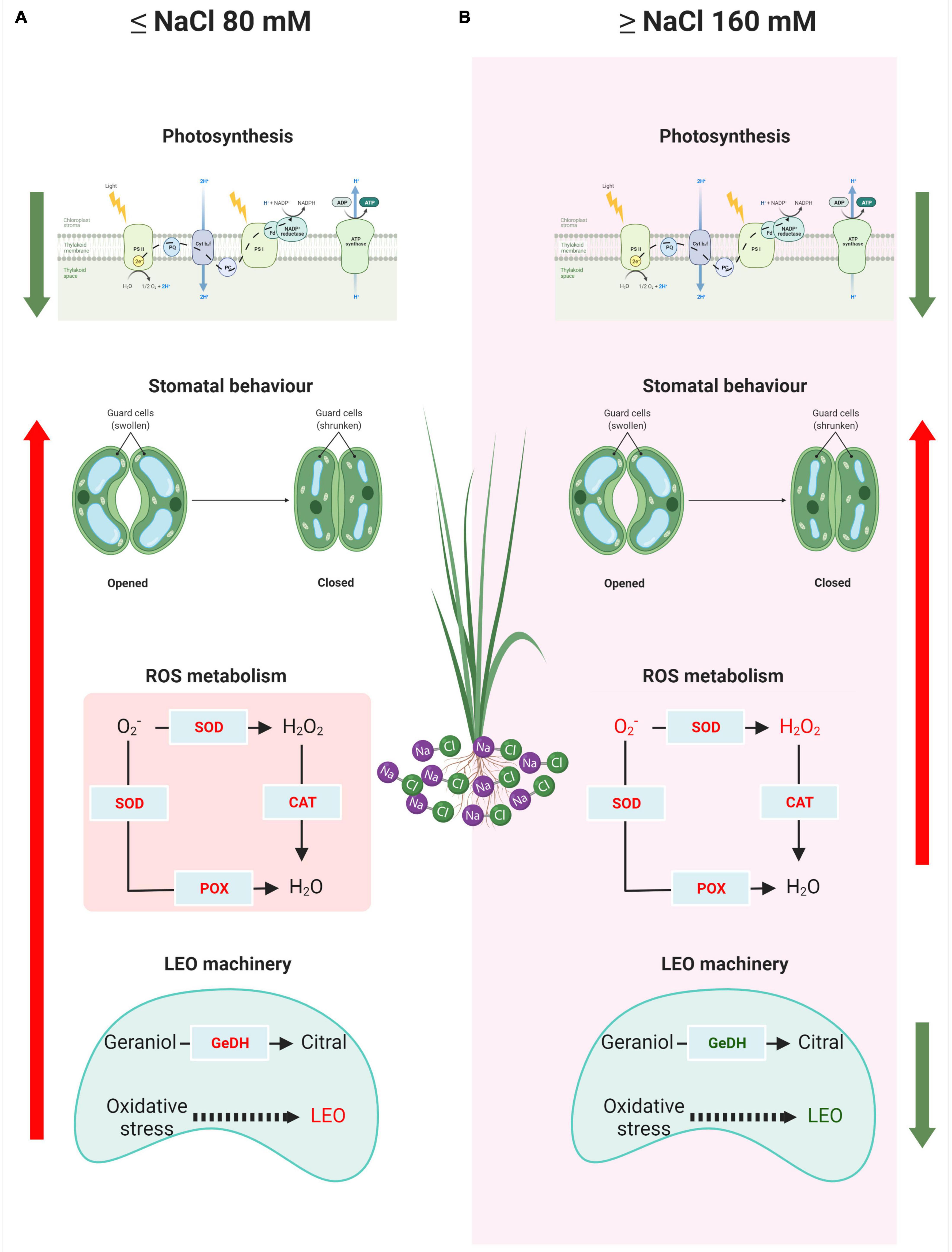
Figure 2. Dissimilar response model of a lemongrass plant at low (A) and high (B) salt concentration. The upward arrows indicate upregulation while green downward arrows signify downregulation in the process. O2–, superoxide ion; H2O2, hydrogen peroxide content; CAT, catalase activity; POX, peroxidase activity; SOD, superoxide dismutase activity; GeDH, geraniol dehydrogenase activity; LEO, lemongrass essential oil.
Experimental Results
Lemongrass Growth and Development Under Salt Stress
Lemongrass growth and development were compromised during all the salt concentrations. However, under slightly to moderate salinity (≤80 mM), lemongrass plants retained most of their height, biomass, and leaf area as compared to control plants (Figures 3A–C). The lemongrass retained about 89% of its height under moderate salinity whereas this percentage was observed to be about 90 and 88 for dry weight and leaf area measurements, respectively. Nevertheless, at the advent of the highly saline regime, plant height was reduced by 21% over the control. Similarly, dry weight and leaf area were restricted by 22 and 20% over the control under NaCl 160 mM. The maximised reduction in all the studied variables was observed with NaCl 240 mM including plant height (50%), dry weight (47%), and leaf area (43%) when compared with the control.
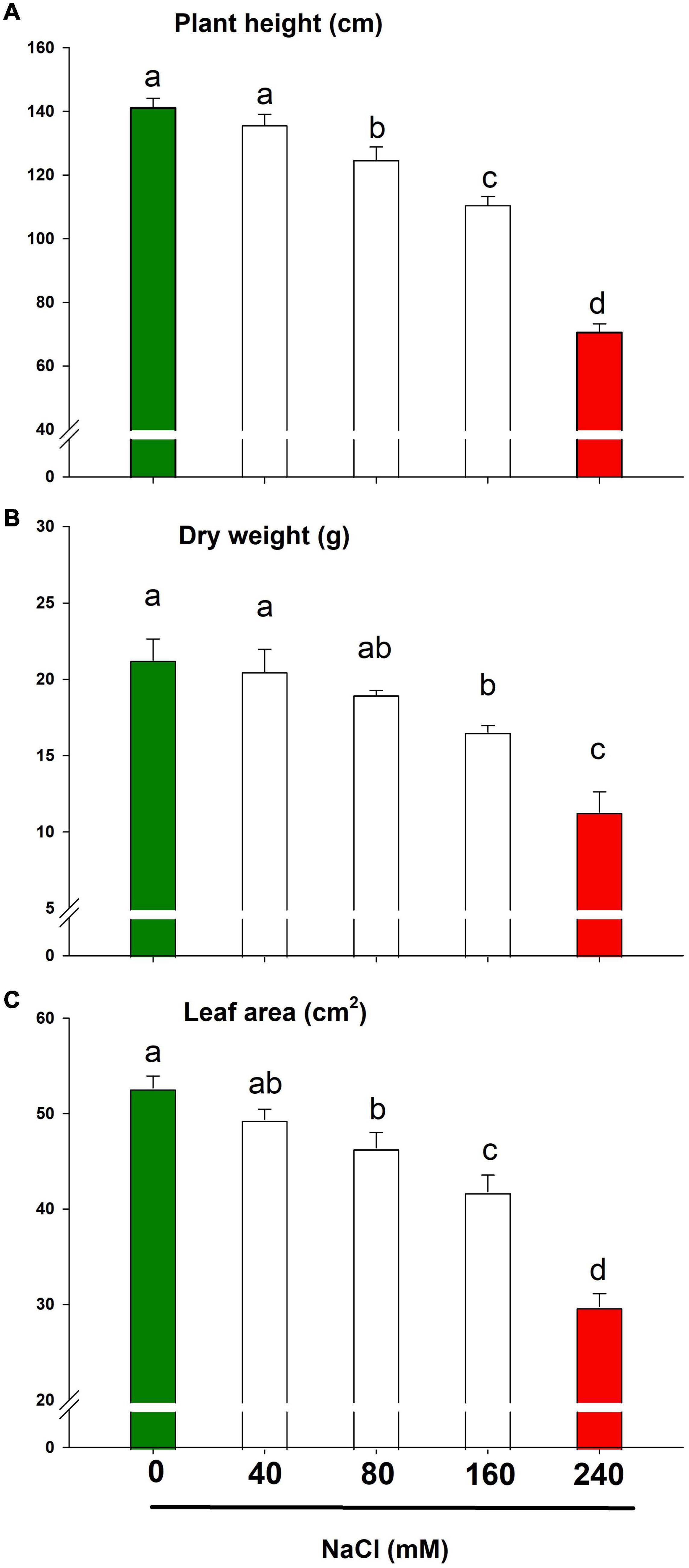
Figure 3. Growth parameters of lemongrass plant under the different salt regimes (0, 40, 80, 160, and 240 mM NaCl) depicting plant height (A), dry weight (B), and leaf area (C). Each bar represents mean ± SE (n = 3). Means followed by the same letter(s) do not differ by LSD test at 5% probability level (α = 0.05). The colors are just visual ques for readers to quickly distinguish between control and most stressed treatment.
Photosynthetic Machinery During Salinity
The chlorophyll content and chlorophyll fluorescence are important markers for the photosynthetic health of a plant. In the present study, the retarded growth traits were underpinned by collapsed photosynthesis in lemongrass leaves. The result showed significant downregulation in photosynthetic pigment and fluorescence with each salt concentration (Figures 4A,B). Nevertheless, chlorophyll content was diminished by about 43% over the control by NaCl 240 mM. The same treatment caused the maximised distortion of 27% in chlorophyll fluorescence over the control plants.
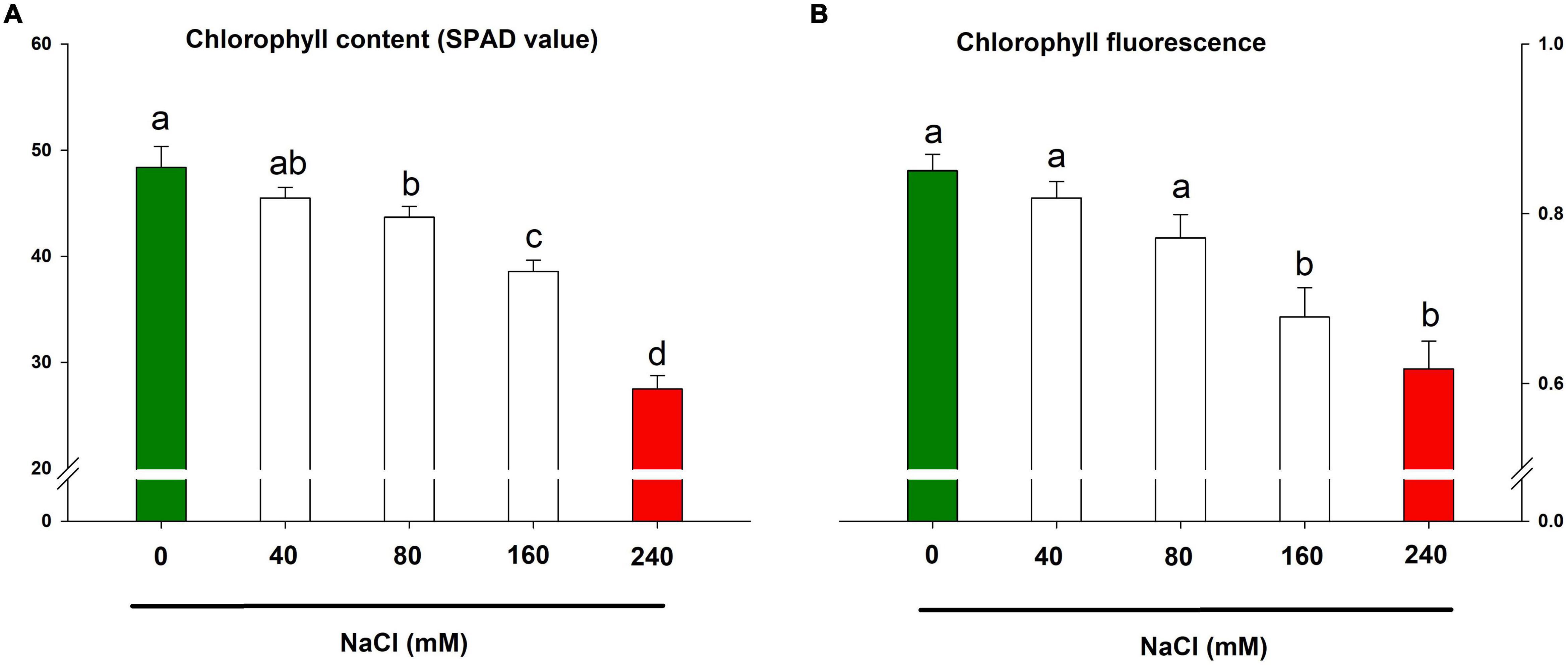
Figure 4. Impact of NaCl concentrations (0, 40, 80, 160, and 240 mM) on chlorophyll content (A) and maximum quantum efficiency of PSII photochemistry (B) of lemongrass plants. Each bar represents mean ± SE (n = 3). Means followed by the same letter(s) do not differ by LSD test at 5% probability level (α = 0.05). The colors are just visual ques for readers to quickly distinguish between control and most stressed treatment.
Lemongrass Gas Exchange and Salt Stress
Lemongrass gas exchange was determined in terms of net photosynthetic rate, stomatal conductance, and transpiration rate. Although a slightly saline regime (NaCl 40 mM) did not bring any significant (α = 0.05) changes in the net photosynthetic and transpiration rate, higher salt content restricted stomatal behaviour to a minimum (Figures 5A–C). The extremely saline regime, i.e., NaCl 240 mM limited PN by about 43% over the control while gs was reduced by about 45%. Similarly, the values for E were reduced by 27 and 56% with 160 mM and 240 mM of NaCl, respectively.
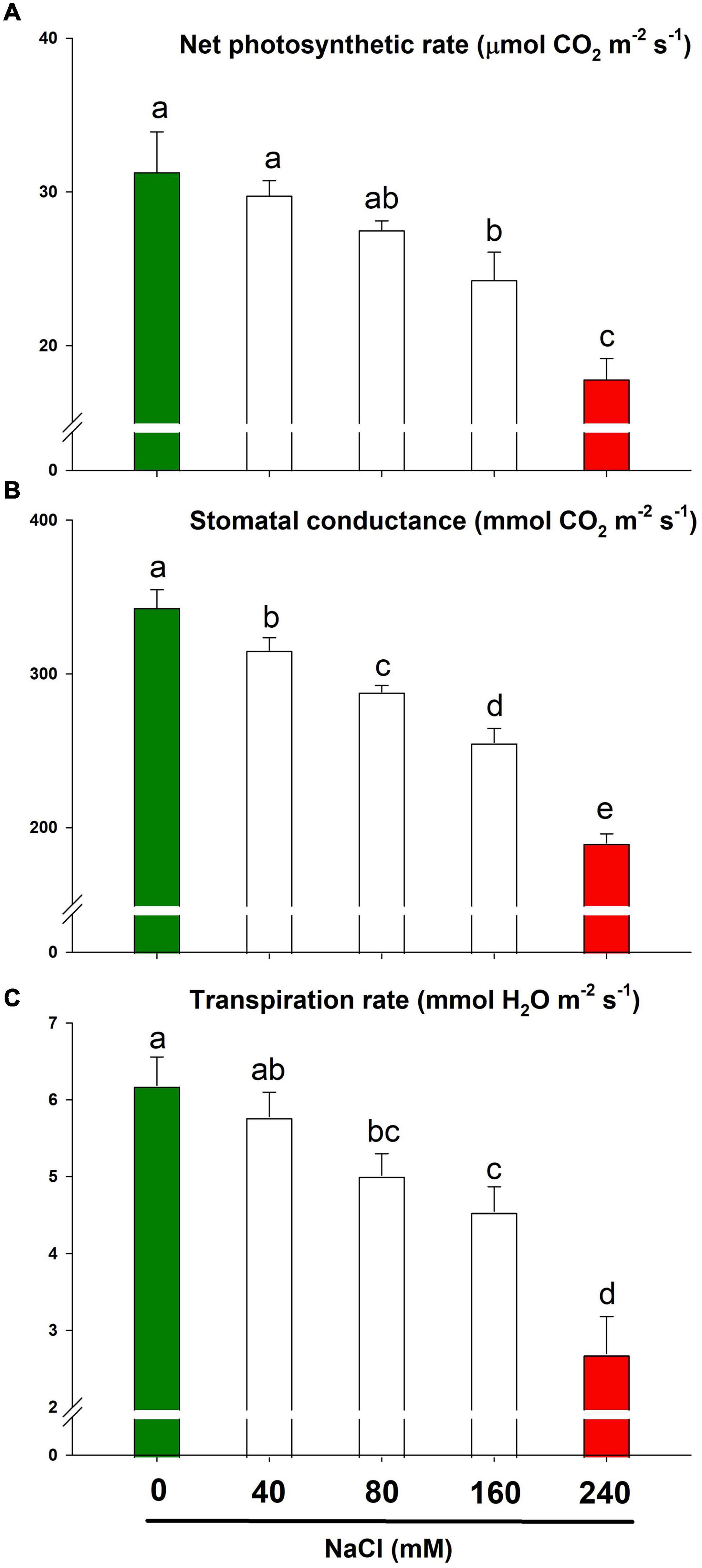
Figure 5. The gas exchange response of lemongrass to NaCl concentrations (0, 40, 80, 160, and 240 mM) with the focus on net photosynthetic rate (A), stomatal conductance (B), and transpiration rate (C). Each bar represents mean ± SE (n = 3). Means followed by the same letter(s) do not differ by LSD test at 5% probability level (α = 0.05). The colors are just visual ques for readers to quickly distinguish between control and most stressed treatment.
Salt-Induced Oxidative Damage
The H2O2 and TBARS contents are the known marker for oxidative stress and lipid peroxidation and thus, in turn, cellular damage. Both H2O2 and TBARS contents were positively correlated with increasing salt concentrations allowing aggravated oxidative stress and lipid peroxidation with higher doses, e.g., 160 mM and 240 mM (Figures 6A,B). The highest accumulation of H2O2 and TBARS contents was caused by NaCl 240 mM application loosely followed by NaCl 160 mM.
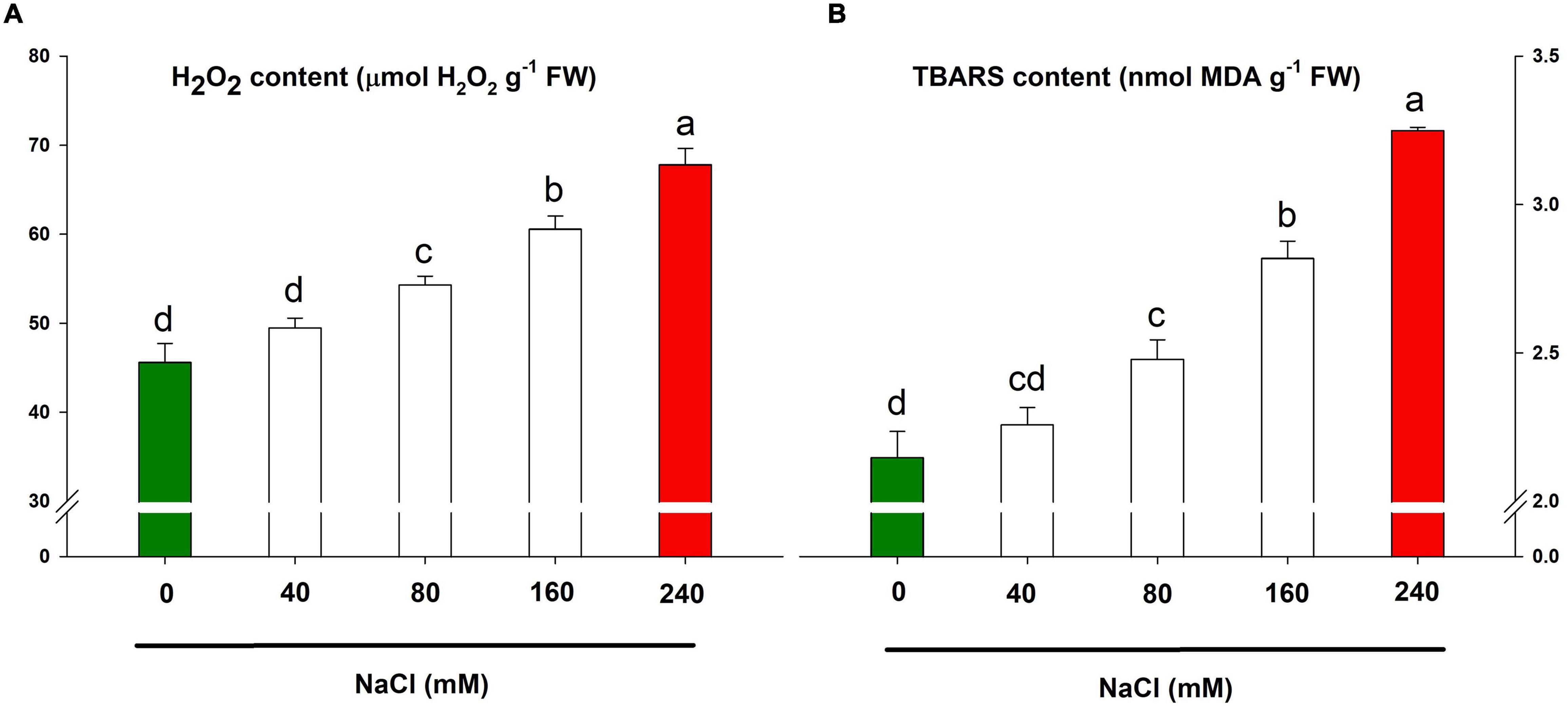
Figure 6. Oxidative stress markers for assessing the salt-sensitivity in lemongrass plants. H2O2 content (A) and TBARS content (B) are depicted here. Each bar represents mean ± SE (n = 3). Means followed by the same letter(s) do not differ by LSD test at 5% probability level (α = 0.05). The colors are just visual ques for readers to quickly distinguish between control and most stressed treatment.
Lemongrass Defence System for Salt Tolerance
The innate defence system of lemongrass exhibited pronounced antioxidative activities to resist salt-induced oxidative shock. However, it is apparent from Figures 7A–C that the lemongrass antioxidative system was not enough to tolerate higher salinity levels (particularly 160 and 240 mM). Thus, plants suffered the severest and had the lowest growth and development during these treatments. The antioxidant system was most intensified during NaCl 240 mM with an increase of about 47, 43, and 47% in CAT, POX, and SOD activities, respectively, over the control.
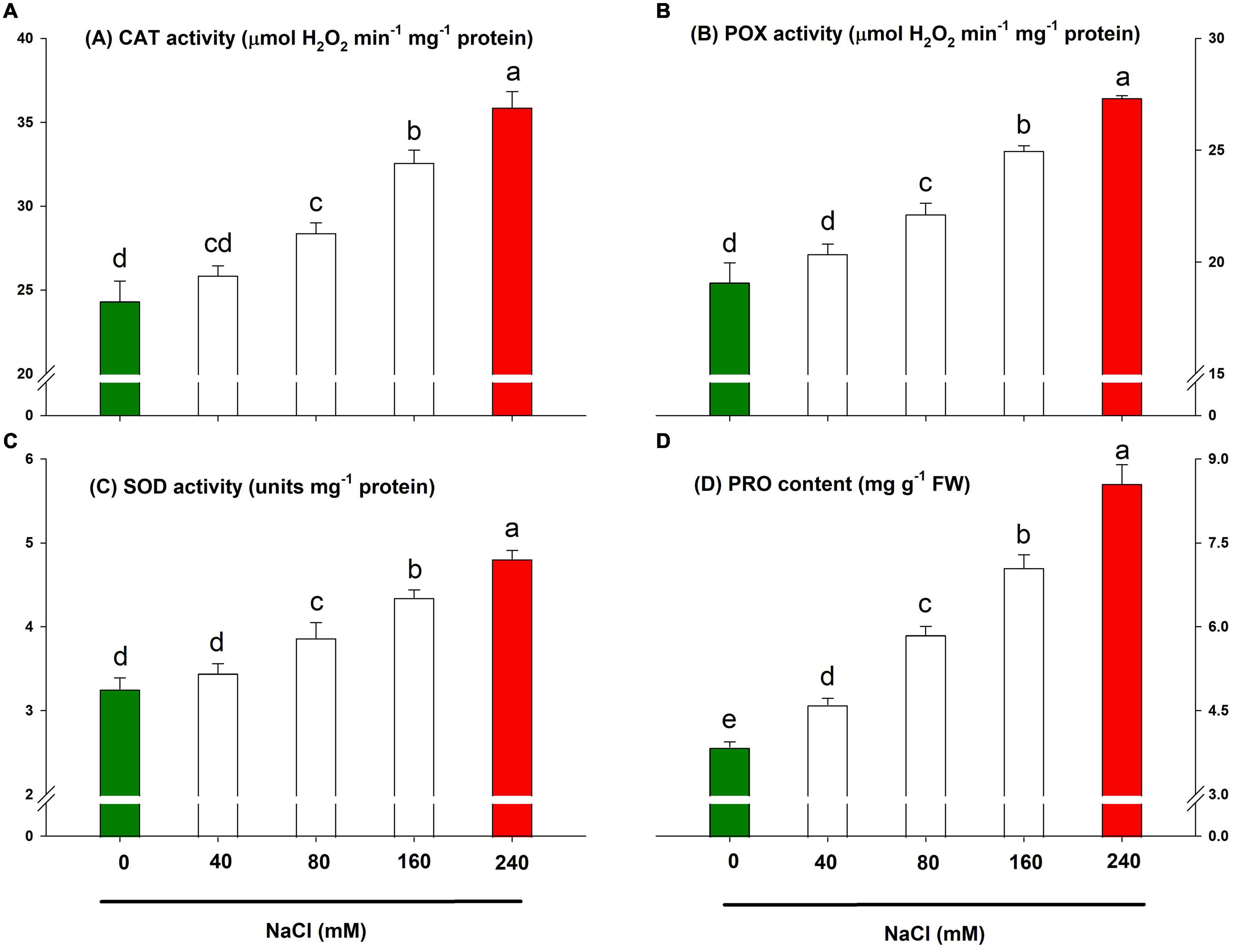
Figure 7. Lemongrass defence system and salinity stress. The activities of enzymatic antioxidants such as catalase (A), peroxidase (B), and superoxide dismutase (C), and the content of osmolyte proline (D) are depicted here. Each bar represents mean ± SE (n = 3). Means followed by the same letter(s) do not differ by LSD test at 5% probability level (α = 0.05). The colors are just visual ques for readers to quickly distinguish between control and most stressed treatment.
Parallel to enzymatic antioxidants, the osmolyte content rose with increasing salt severity. PRO, a key osmolyte, was quantified to appraise osmoprotection during salinity stress in the present experiment. Although each NaCl treatment produced magnified PRO content, the highest upgradation was brought by NaCl 240 mM application with an increase of about 122% in PRO content over the control (Figure 7D).
Essential Oil Machinery Under Salinity
The activity of GeDH, a key enzyme of the essential oil biosynthetic pathway in lemongrass, exhibited a differential response to saline treatments in comparison to earlier mentioned parameters. The salt concentrations of 40 and 80 mM slightly upregulated the enzyme activity by about 3 and 7%, respectively (Figure 8A). The upregulated enzyme increased essential oil content by about 8 and 17% (Figure 8B). Nevertheless, the higher salt concentrations (160 and 240 mM) reduced the enzyme activity by about 22 and 40%, respectively. The heavy restriction of NaCl 240 mM over the enzymatic profile pointed to the salt severity on the essential oil biosynthesis in lemongrass plants (Figures 8A,B).
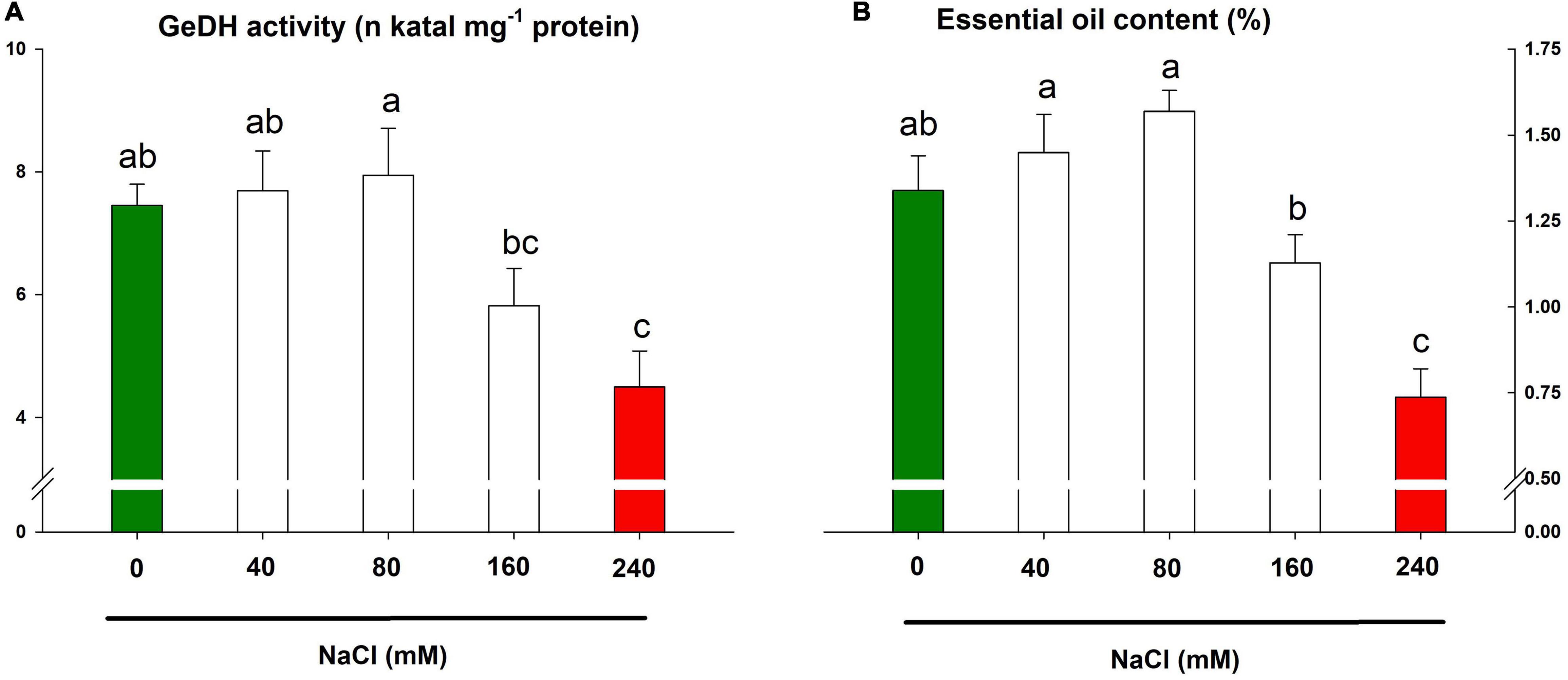
Figure 8. Lemongrass essential oil machinery under salinity stress. The impact of different salt concentrations (0, 40, 80, 160, and 240 mM) was observed on geraniol dehydrogenase (GeDH) activity (A) and essential oil content (B) of lemongrass plants. Each bar represents mean ± SE (n = 3). Means followed by the same letter(s) do not differ by LSD test at 5% probability level (α = 0.05). The colors are just visual ques for readers to quickly distinguish between control and most stressed treatment.
This was evident with the drop in essential oil content with salt severity. The reduced photosynthesis, development, and enzymatic activity compromised oil productivity in lemongrass. Therefore, the bottom values were obtained with NaCl 240 mM when it perished oil content by about 45% over the control.
Moreover, a strong correlation was observed among the studied parameters pertaining to the growth, development, and yield of lemongrass plants during all the five salt regimes. A heatmap (Figure 9) was drawn based on Pearson correlation values. All correlation pairings were significant (α = 0.05) except transpiration with geraniol dehydrogenase activity and transpiration with essential oil content. The correlation matrix revealed a strong dependency of lemongrass photosynthetic machinery upon the innate defence system (CAT, POX, SOD, and PRO) of the plant. Moreover, geraniol dehydrogenase activity and essential oil content correlate positively with photosynthetic machinery and negatively with the antioxidant defence system. Furthermore, principal component analysis (PCA) for the various parameters of growth, development, and productivity was carried out. The first two principal components captured 92% of total variability, thus further principal components were dismissed. PCA results showed similar covariance between the traits as shown in correlation analysis (Figure 10). The 95% confidence ellipses within the PCA scatterplot showed that there was no overlap between the treatments based on their overall performance (Figure 11). The treatment exposed to 80 mM (T2) is the only treatment entirely located in the third quadrant of the scatter plot (Figure 11). This position is driven by the high activity of GeDH, and high essential oil content coupled with high photosynthetic performance and growth (Figure 10).
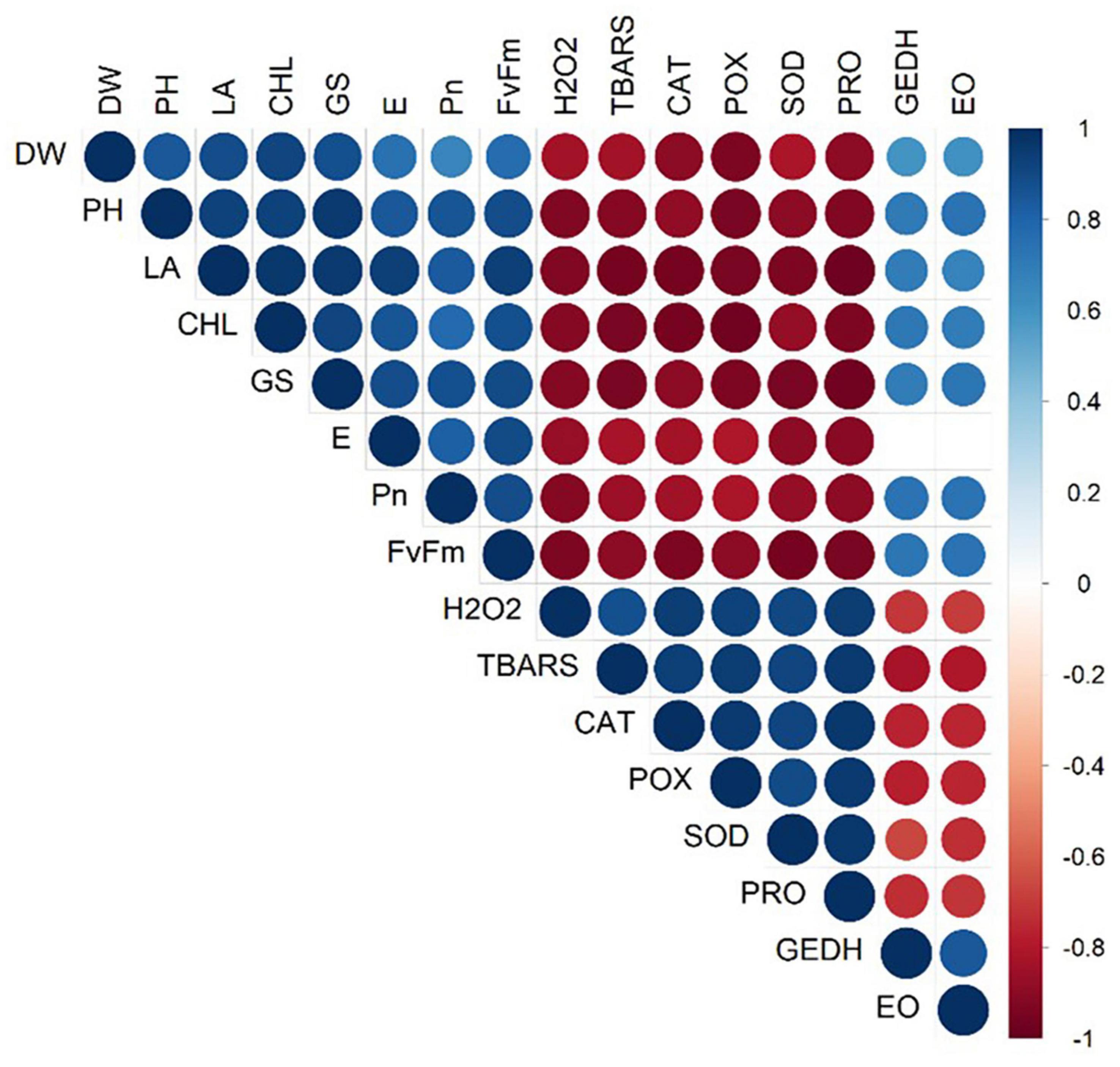
Figure 9. Pearson correlation index heatmap of all tested parameters under different saline regimes (0, 40, 80, 160, and 240 mM of NaCl). The positive correlation is shown with blue colour while the red colour represents a negative correlation. Non-significant correlations at α = 0.05 are excluded from the heatmap. DW, dry weight; PH, plant height; LA, leaf area; CHL, chlorophyll content; GS, stomatal conductance; E, transpiration rate; Pn, net photosynthetic rate; Fv/Fm, quantum yield; H2O2, hydrogen peroxide content; TBARS, thiobarbituric acid reactive substances content; CAT, catalase activity; POX, peroxidase activity; SOD, superoxide dismutase activity; PRO, proline content; GEDH, geraniol dehydrogenase activity; EO, essential oil content.
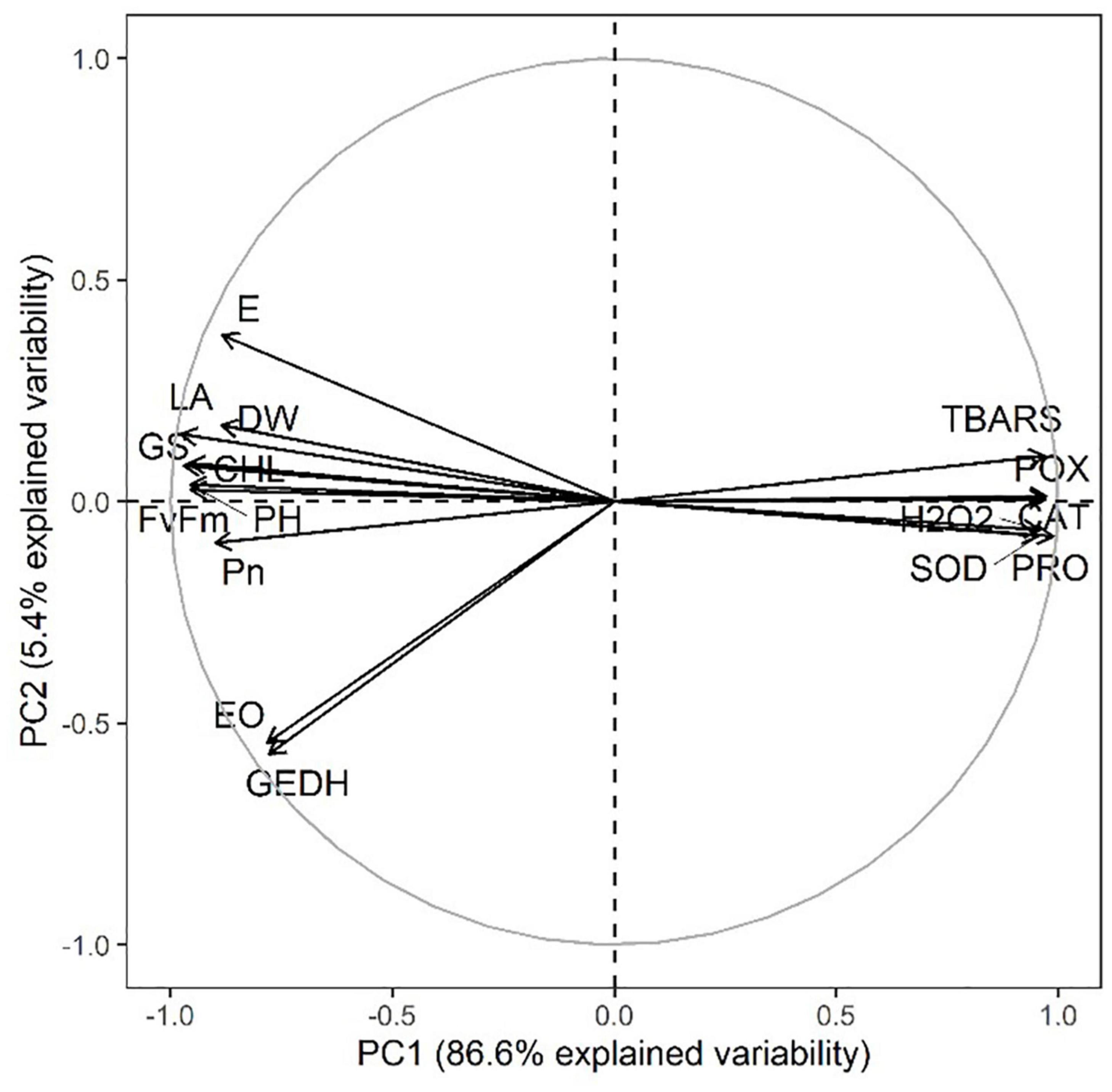
Figure 10. PCA variables factor map of all tested parameters and all five treatments. DW, dry weight; PH, plant height; LA, leaf area; CHL, chlorophyll content; GS, stomatal conductance; E, transpiration rate; Pn, net photosynthetic rate; Fv/Fm, quantum yield; H2O2, hydrogen peroxide content; TBARS, thiobarbituric acid reactive substances content; CAT, catalase activity; POX, peroxidase activity; SOD, superoxide dismutase activity; PRO, proline content; GEDH, geraniol dehydrogenase activity; EO, essential oil content.
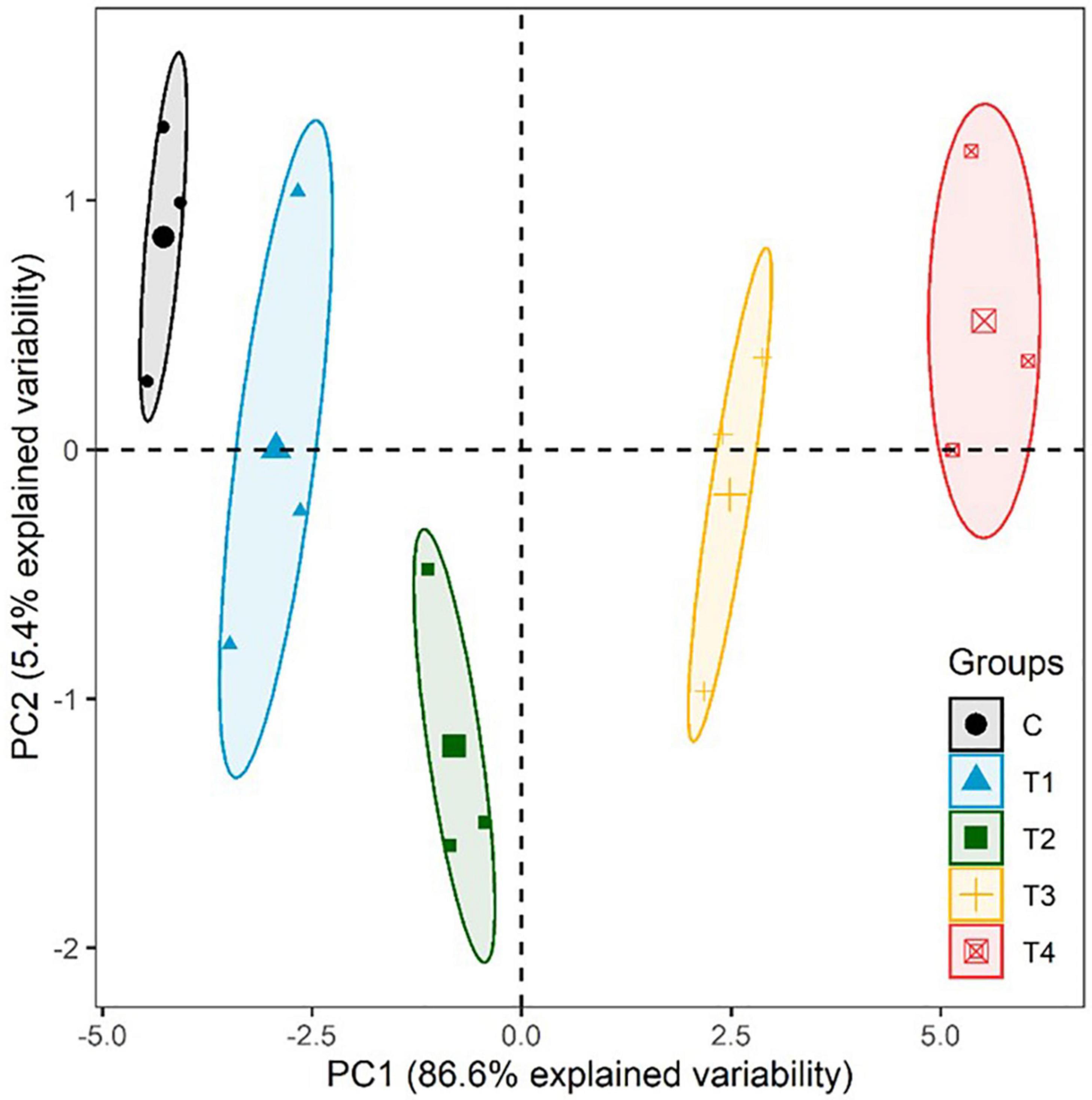
Figure 11. PCA scatter plot with centroids and 95% confidence ellipses. Principal components were derived from all tested parameters and treatments. C, 0 mM NaCl; T1, 40 mM NaCl; T2, 80 mM NaCl; T3, 160 mM NaCl; T4, 240 mM NaCl.
Discussion
Soil salinity is an acute threat that is continuously claiming irrigable lands around the globe (Qadir et al., 2014). Nevertheless, the damaging effects of salinity can vary depending on its concentration and plant adaptability (Munns and Tester, 2008). Considering a growing market for lemongrass and its essential oil (Haque et al., 2018; Mukarram et al., 2021a,b), the present experiment traces the salt-sensitivity of lemongrass and the efficacy of its defence system against salinity.
Lemongrass Growth and Salinity
Lemongrass growth was determined in terms of plant height, dry weight, and leaf area. All the salt treatments (40, 80, 160, and 240 mM of NaCl) reduced plant height, dry weight, and leaf area in a dose-dependent manner where the highest salt concentration posed the severest damage. Although the damage occurred in the height and dry weight of the plant at slightly and moderate salinity, the reduction was below 20%. Moderate resistance of lemongrass under salinity stress reflected in stable biomass production was also observed by Ullah et al. (2020). However, with the advent of high and extreme salinity, the height and dry weight of the plant was restricted up to 50%. Similar implications of salinity were observed in many other crops including rice (Kibria et al., 2017), wheat (Saddiq et al., 2021), barley (Zeeshan et al., 2020), maize (Hessini et al., 2019), tomato (Diouf et al., 2018), and potato (Chourasia et al., 2021).
Salt Implications on Lemongrass Physiology and Its Feedback Mechanism
Salt stress can restrict photosynthesis and associated phenomena that stunts growth and plant development. Photosynthetic retardation is supported by multiple stomatal and non-stomatal restrictions (Kiani-Pouya et al., 2020; Pan et al., 2020). It was noticed that lemongrass, like many other glycophytes, closed their stomata during high (NaCl 160 mM) and extreme (NaCl 240 mM) salinity as a feedback mechanism to minimise the transpiration loss. Nevertheless, it seems pertinent that extended stomatal closure would reduce carbon dioxide intake and, subsequently, carbon assimilation. Similar implications were observed in the present experiment where growing salinity menaced PN and E in lemongrass plants. Furthermore, salt-affected lemongrass plants might have higher chlorophyllase activity inhibiting chlorophyll biosynthesis and altering chloroplast ultrastructure through oxidative peroxidation (Flexas et al., 2004; Chaves et al., 2009; Gupta and Pandey, 2020). Further, higher ROS content could also have detrimental effects on lemongrass photosynthetic machinery through an intricate signalling pathway (Foyer, 2018). This might have resulted in reduced chlorophyll content and chlorophyll fluorescence as was observed during different salt regimes.
Increasing salt concentrations in the rhizosphere can limit water and mineral uptake and create an osmotic imbalance. The plant chooses its survival over the growth during inefficient water and nutrient uptake. To achieve osmotic balance, plants direct the energy acquired (from nutrients, water, and photosynthates) to accelerate the production of osmolytes including proline, soluble sugars, polyamines, etc.
Additionally, a general trend of elicitation was noticed in CAT, POX, and SOD activities along with PRO content. The upregulated antioxidant profile and osmolyte during stress is a common defence mechanism in many plants to render enhanced protection against stress-induced oxidative and osmotic damage (Das and Roychoudhury, 2014; Foyer, 2018; Noctor et al., 2018). In addition to salinity stress, higher CAT activity in Lemongrass was also observed during drought (Mirzaei et al., 2020), heavy metal stress (Patra et al., 2019), and seasonal heat (Aziz et al., 2014). Moreover, lemongrass exposed to soil salinity stress increased its SOD activity (Rehman et al., 2022). Similarly, an increase in PRO content in lemongrass was observed under heavy metal stress and drought (Patra et al., 2019; Mirzaei et al., 2020).
Salinity and Essential Oil Biosynthesis
Since lemongrass oil has antioxidant and antibiological activities, its oil concentration usually rises as a defence mechanism at the onset of a stressful environment (Singh and Anwar, 1985; Mirzaei et al., 2020; Mukarram et al., 2021b). A similar trend was observed during slightly (40 mM) and moderately (NaCl 80 mM) saline treatments even though the differences were not significant. However, at higher salt concentrations (NaCl 160 and 240 mM), LEO machinery might have been exhausted, as evident by the noticeable decrease in lemongrass oil over the control. Similarly, exposure of lemongrass to 150 mM NaCl led to a significant reduction of essential oil content in two cultivars (Idrees et al., 2011). The transcripts related to LEO biosynthesis such as CfADH1, CfADH2a-b, CfAAT3, and CfALDH, are responsible for GeDH activity (Meena et al., 2016). Since the GeDH enzyme influences the essential oil biosynthesis in lemongrass, it is feasible to assume these transcripts were downregulated under increased salinity resulting in reduced GeDH activity as we observed with 160 and 240 mM of NaCl. The downgraded enzyme activity can relate to the decreased essential oil content obtained in the present study during the extreme salt regime. However, the essential oil productivity during moderate salinity suggests a viable LEO machinery.
Figure 2 presents the comprehensive understanding of the salt-sensitivity of lemongrass plants devised during the present study. The salt concentration of 80 mM or below imposes photosynthetic restrictions in the plant, however, the closing of stomata, antioxidant activity, and essential oil production are elicited. Lower NaCl concentrations (≤80 mM) induce oxidative stress through ROS accumulation, however, lemongrass antioxidants are also overexpressed at this point for ROS scavenging. This discourages further stress build-up and aids cellular homoeostasis (Figure 2A). On the other hand, higher salinity (≥NaCl 160 mM) compromises photosynthetic assembly and essential oil biosynthesis which result in reduced photosynthates and oil production. Furthermore, extremely low transpiration rate and stomatal conductance seem to have active participation in the salt-induced productivity loss. Although antioxidants were highly active at higher salt concentrations, the accumulated H2O2 and TBARS content suggest an inefficient defence system in lemongrass during higher salt concentrations (Figure 2B).
Conclusion
The acute threat of salinity stress is ever-present. Depending on the severity, it poses a reduction in crop growth, development, and productivity, or plant death. Further, different crops respond to salinity differently as a product of their susceptibility. Given the commercial importance of lemongrass, the present experiment was conducted to explore the susceptibility level of lemongrass plants to different salt severities. The result of the study suggests that the lemongrass is a “moderately salt-sensitive” crop. Metabolomic approaches revealed that it can maintain growth and essential oil synthesis under moderate salinity stress (NaCl 80 mM) via upregulation in ROS and antioxidants metabolism. Nevertheless, higher salinity stress (≥NaCl 160 mM) inhibits photosynthesis due to PSII retardation and lowers chlorophyll regeneration in addition to restricting stomatal conductance. Furthermore, the innate defence system of the plant comprising CAT, POX, and SOD antioxidants and osmolyte PRO also struggles to render an efficient antioxidative defence and osmoprotection amidst ROS overaccumulation. This leads to significantly lower growth and essential oil accumulation. Further, the authors suggest the lemongrass for reclamation of saline lands considering that the other members of the Poaceae family are more sensitive to salinity and cannot grow well in such areas. The salinity tolerance threshold of major crops such as rice (30 mM), wheat (60 mM), barley (60 mM), sorghum (65–70 mM), sugarcane (15–20 mM), and maize (15–20 mM) is significantly lower than the lemongrass (80 mM) (Maas and Grattan, 1999). Additionally, the lemongrass holds a special reference to the Indian economy given the exponential growth in lemongrass export (>1250%) within the past two decades (Mukarram et al., 2021b). Therefore, further studies could be relevant in extending the cultivation of lemongrass crops in reclaiming the salt-affected lands (≤80 mM).
Data Availability Statement
The raw data supporting the conclusions of this article will be made available by the authors, without undue reservation.
Author Contributions
MK: conceptualisation, validation, supervision, and project administration. MM and MK: methodology. MM and PP: software and formal analysis. MM and AZ: investigation. DK and MK: resources, writing – review and editing, visualisation, and funding acquisition. MM, AZ, and PP: data curation and writing – original draft preparation. All authors have read and agreed to the published version of the manuscript.
Funding
MM was supported by the Slovak Research and Development Agency number APVV-19-0035. DK was supported from the Slovak Grant Agency for Science, No. VEGA 1/0535/20.
Conflict of Interest
The authors declare that the research was conducted in the absence of any commercial or financial relationships that could be construed as a potential conflict of interest.
Publisher’s Note
All claims expressed in this article are solely those of the authors and do not necessarily represent those of their affiliated organizations, or those of the publisher, the editors and the reviewers. Any product that may be evaluated in this article, or claim that may be made by its manufacturer, is not guaranteed or endorsed by the publisher.
Acknowledgments
We acknowledge Farheen Anjum (Department of English, AMU, Aligarh) for her kind help with the visual representation of the data and Saif Ali Khan (Department of Statistics and Operations Research, AMU, Aligarh) for his kind assistance with the data analyses in the earlier version of the manuscript. MM thanks NSP (SAIA), Slovakia for financing his research stay in Slovakia.
References
Aftab, K., Ali, M., Aijaz, P., Beena, N., Gulzar, H. J., Sheikh, K., et al. (2011). Determination of different trace and essential element in lemon grass samples by x-ray fluorescence spectroscopy technique. Int. Food Res. J. 18, 265–270.
Ashraf, M. (2009). Biotechnological approach of improving plant salt tolerance using antioxidants as markers. Biotechnol. Adv. 27, 84–93. doi: 10.1016/j.biotechadv.2008.09.003
Aziz, A., Wahid, A., and Farooq, M. (2014). Leaf age and seasonality determines the extent of oxidative stress and induction of antioxidants in lemongrass. Pak. J. of Agric. Sci. 51, 657–664.
Bates, L. S., Waldren, R. P., and Teare, I. D. (1973). Rapid determination of free proline for water-stress studies. Plant Soil 39, 205–207. doi: 10.1007/BF00018060
Beauchamp, C., and Fridovich, I. (1971). Superoxide dismutase: Improved assays and an assay applicable to acrylamide gels. Anal. Biochem. 44, 276–287. doi: 10.1016/0003-2697(71)90370-8
Cakmak, I., and Horst, W. J. (1991). Effect of aluminium on lipid peroxidation, superoxide dismutase, catalase, and peroxidase activities in root tips of soybean (Glycine max). Physiol. Plant. 83, 463–468. doi: 10.1111/j.1399-3054.1991.tb00121.x
Chaves, M. M., Flexas, J., and Pinheiro, C. (2009). Photosynthesis under drought and salt stress: regulation mechanisms from whole plant to cell. Ann. Bot. 103, 551–560. doi: 10.1093/aob/mcn125
Chourasia, K. N., Lal, M. K., Tiwari, R. K., Dev, D., Kardile, H. B., Patil, V. U., et al. (2021). Salinity stress in potato: understanding physiological, biochemical and molecular responses. Life 11:545. doi: 10.3390/LIFE11060545
Das, K., and Roychoudhury, A. (2014). Reactive oxygen species (ROS) and response of antioxidants as ROS-scavengers during environmental stress in plants. Front. Environ. Sci. 2:53. doi: 10.3389/fenvs.2014.00053
Dhinesh, B., Lalvani, J. I. J., Parthasarathya, M., and Annamalaia, K. (2016). An assessment on performance, emission and combustion characteristics of single cylinder diesel engine powered by Cymbopogon flexuosus biofuel. Energy Convers. Manag. 117, 466–474. doi: 10.1016/j.enconman.2016.03.049
Diouf, I. A., Derivot, L., Bitton, F., Pascual, L., and Causse, M. (2018). Water deficit and salinity stress reveal many specific QTL for plant growth and fruit quality traits in tomato. Front. Plant Sci. 9:279. doi: 10.3389/FPLS.2018.00279
Flexas, J., Bota, J., Loreto, F., Cornic, G., and Sharkey, T. D. (2004). Diffusive and metabolic limitations to photosynthesis under drought and salinity in C3 plants. Plant Biol. 6, 269–279. doi: 10.1055/S-2004-820867/ID/90
Flowers, T. J., and Colmer, T. D. (2008). Salinity tolerance in halophytes. New Phytol. 179, 945–963. doi: 10.1111/j.1469-8137.2008.02531.x
Foyer, C. H. (2018). Reactive oxygen species, oxidative signaling and the regulation of photosynthesis. Environ. Exp. Bot. 154, 134–142. doi: 10.1016/j.envexpbot.2018.05.003
Gaba, J., Bhardwaj, G., and Sharma, A. (2020). “Lemongrass,” in Antioxidants in Vegetables and Nuts - Properties and Health Benefits, eds G. S. Nayik and A. Gull (Singapore: Springer).
Guenther, E. (1972). The essential oils: history, origin in plants, production. Analysis 1, 147–151.
Gupta, S., and Pandey, S. (2020). Enhanced salinity tolerance in the common bean (Phaseolus vulgaris) plants using twin ACC deaminase producing rhizobacterial inoculation. Rhizosphere 16:100241. doi: 10.1016/J.RHISPH.2020.100241
Haque, A. N. M. A., Remadevi, R., and Naebe, M. (2018). Lemongrass (Cymbopogon): a review on its structure, properties, applications and recent developments. Cellulose 25, 5455–5477. doi: 10.1007/s10570-018-1965-2
Hessini, K., Issaoui, K., Ferchichi, S., Saif, T., Abdelly, C., Siddique, K. H., et al. (2019). Interactive effects of salinity and nitrogen forms on plant growth, photosynthesis and osmotic adjustment in maize. Plant Physiol. Biochem. 139, 171–178. doi: 10.1016/J.PLAPHY.2019.03.005
Idrees, M., Naeem, M., Aftab, T., and Khan, M. (2011). Salicylic acid mitigates salinity stress by improving antioxidant defence system and enhances vincristine and vinblastine alkaloids production in periwinkle [Catharanthus roseus (L.) G. Don]. Acta Physiol. Plant. 33, 987–999.
Kiani-Pouya, A., Rasouli, F., Rabbi, B., Falakboland, Z., Yong, M., Chen, Z. H., et al. (2020). Stomatal traits as a determinant of superior salinity tolerance in wild barley. J. Plant Physiol. 245:153108. doi: 10.1016/J.JPLPH.2019.153108
Kibria, M. G., Hossain, M., Murata, Y., and Hoque, M. A. (2017). Antioxidant defense mechanisms of salinity tolerance in rice genotypes. Rice Sci. 24, 155–162. doi: 10.1016/J.RSCI.2017.05.001
Kumar, K. B., and Khan, P. (1982). Peroxidase and polyphenol oxidase in excised ragi (Eleusine corocana cv PR 202) leaves during senescence. Indian J. Exp. Biol. 20, 412–416.
Kuo, T. M., Warner, R. L., and Kleinhofs, A. (1982). In vitro stability of nitrate reductase from barley leaves. Phytochemistry 21, 531–533. doi: 10.1016/0031-9422(82)83134-8
Maas, E. V., and Grattan, S. R. (1999). Crop yields as affected by salinity. Agricultural Drainage 38, 55–108.
Machado, R., and Serralheiro, R. (2017). Soil salinity: effect on vegetable crop growth. management practices to prevent and mitigate soil salinization. Horticulturae 3:30. doi: 10.3390/horticulturae3020030
Meena, S., Kumar, S. R., Venkata Rao, D. K., Dwivedi, V., Shilpashree, H. B., Rastogi, S., et al. (2016). De novo sequencing and analysis of lemongrass transcriptome provide first insights into the essential oil biosynthesis of aromatic grasses. Front. Plant Sci. 7:1129. doi: 10.3389/fpls.2016.01129
Mirzaei, M., Moghadam, L. A., Hakimi, L., and Danaee, E. (2020). Plant growth promoting rhizobacteria (PGPR) improve plant growth, antioxidant capacity, and essential oil properties of lemongrass (Cymbopogon citratus) under water stress. Iran. J. Plant Physiol. 10, 3155–3166.
Mukarram, M., Khan, M. M. A., Zehra, A., Choudhary, S., Naeem, M., and Aftab, T. (2021a). “Biosynthesis of lemongrass essential oil and the underlying mechanism for its insecticidal activity,” in Medicinal and Aromatic Plants, eds T. Aftab and K. R. Hakeem (Cham: Springer).
Mukarram, M., Choudhary, S., Khan, M. A., Poltronieri, P., Khan, M. M. A., Ali, J., et al. (2021b). Lemongrass essential oil components with antimicrobial and anticancer activities. Antioxidants 11:20. doi: 10.3390/antiox11010020
Mukarram, M., Khan, M., Uddin, M., and Corpas, F. J. (2021c). Irradiated chitosan (ICH): an alternative tool to increase essential oil content in lemongrass (Cymbopogon flexuosus). Acta Physiol. Plant. 44, 1–15. doi: 10.1007/S11738-021-03335-W
Mukarram, M., Khan, M. M. A., and Corpas, F. J. (2021d). Silicon nanoparticles elicit an increase in lemongrass (Cymbopogon flexuosus (Steud.) Wats) agronomic parameters with a higher essential oil yield. J. Hazard. Mater. 412:125254. doi: 10.1016/j.jhazmat.2021.125254
Mukarram, M., Choudhary, S., Kurjak, D., Petek, A., and Khan, M. M. A. (2021e). Drought: sensing, signalling, effects and tolerance in higher plants. Physiol. Plant. 172, 1291–1300. doi: 10.1111/ppl.13423
Mukarram, M., Mohammad, F., Naeem, M., and Khan, M. (2021f). “Exogenous gibberellic acid supplementation renders growth and yield protection against salinity induced oxidative damage through upregulating antioxidant metabolism in fenugreek (Trigonella foenum-graecum L.),” in Fenugreek, (Singapore: Springer).
Munns, R. (2002). Comparative physiology of salt and water stress. Plant Cell Environ. 25, 239–250. doi: 10.1046/j.0016-8025.2001.00808.x
Munns, R. (2005). Genes and salt tolerance: bringing them together. New Phytol. 167, 645–663. doi: 10.1111/j.1469-8137.2005.01487.x
Munns, R., and Tester, M. (2008). Mechanisms of salinity tolerance. Annu. Rev. Plant Biol. 59, 651–681.
Munns, R., James, R. A., and Läuchli, A. (2006). Approaches to increasing the salt tolerance of wheat and other cereals. J. Exp. Bot. 57, 1025–1043. doi: 10.1093/jxb/erj100
Negrão, S., Schmöckel, S. M., and Tester, M. (2017). Evaluating physiological responses of plants to salinity stress. Ann. Bot. 119, 1–11. doi: 10.1093/aob/mcw191
Noctor, G., Reichheld, J. P., and Foyer, C. H. (2018). ROS-related redox regulation and signaling in plants. Semin. Cell Dev. Biol. 80, 3–12. doi: 10.1016/J.SEMCDB.2017.07.013
Okuda, T., Matsuda, Y., Yamanaka, A., and Sagisaka, S. (1991). Abrupt increase in the level of hydrogen peroxide in leaves of winter wheat is caused by cold treatment. Plant Physiol. 97, 1265–1267. doi: 10.1104/pp.97.3.1265
Pan, T., Liu, M., Kreslavski, V. D., Zharmukhamedov, S. K., Nie, C., Yu, M., et al. (2020). Non-stomatal limitation of photosynthesis by soil salinity. Crit. Rev. Environ. Sci. Technol. 51, 791–825. doi: 10.1080/10643389.2020.1735231
Patra, D. K., Pradhan, C., and Patra, H. K. (2019). Chromium bioaccumulation, oxidative stress metabolism and oil content in lemon grass Cymbopogon flexuosus (Nees ex Steud.) W. Watson grown in chromium rich over burden soil of Sukinda chromite mine, India. Chemosphere 218, 1082–1088.
Pitman, M. G., and Läuchli, A. (2002). “Global impact of salinity and agricultural ecosystems,” in Salinity: Environment - Plants - Molecules, eds A. Läuchli and U. Lüttge (Dordrecht: Kluwer Academic Publishers).
Qadir, M., Quillérou, E., Nangia, V., Murtaza, G., Singh, M., Thomas, R., et al. (2014). Economics of salt-induced land degradation and restoration. Nat. Resour. Forum 38, 282–295. doi: 10.1111/1477-8947.12054
Rehman, Z., Hussain, A., Saleem, S., Khilji, S. A., and Sajid, Z. A. (2022). Exogenous application of salicylic acid enhances salt stress tolerance in lemongrass (Cymbopogon flexuosus Steud. Wats)’, Pak. J. Bot. 54, 371–378.
Richard, L. A. (1954). Diagnosis and Improvement of Saline and Alkali Soils. Agriculture Handbook No. 60. Washington StateDC: United States Department of Agriculture.
Saddiq, M. S., Iqbal, S., Hafeez, M. B., Ibrahim, A. M., Raza, A., Fatima, E. M., et al. (2021). Effect of salinity stress on physiological changes in winter and spring wheat. Agronomy 11:1193. doi: 10.3390/AGRONOMY11061193
Sathiyamoorthi, R., and Sankaranarayanan, G. (2016). Effect of antioxidant additives on the performance and emission characteristics of a DICI engine using neat lemongrass oil–diesel blend. Fuel 174, 89–96. doi: 10.1016/j.fuel.2016.01.076
Shabala, S., and Cuin, T. A. (2008). Potassium transport and plant salt tolerance. Physiol. Plant. 133, 651–669. doi: 10.1111/j.1399-3054.2007.01008.x
Singh, D. V., and Anwar, M. (1985). Effect of soil salinity on herb and oil yield and quality of some Cymbopogon species-Indian Journals. J. Indian Soc. Soil Sci. 33, 362–365. doi: 10.1002/ldr.853
Ullah, M. A., Rasheed, M., and Ishtiaq Hyder, S. (2020). Medicinal plant lemon grass (Cymbopogon citratus) growth under salinity and sodicity. KJFHC 6, 9–15. doi: 10.13106/kjfhc.2020.vol6.no1.9
van Zelm, E., Zhang, Y., and Testerink, C. (2020). Salt tolerance mechanisms of plants. Annu. Rev. Plant Biol. 71, 403–433.
Venkatesan, E. P., Kandhasamy, A., Subramani, L., Sivalingam, A., and Kumar, A. S. (2019). Experimental investigation on lemongrass oil water emulsion in low heat rejection direct ignition diesel engine. J. Test. Eval. 47, 238–255. doi: 10.1520/JTE20170357
Widodo, Patterson, J. H., Newbigin, E., Tester, M., Bacic, A., and Roessner, U. (2009). Metabolic responses to salt stress of barley (Hordeum vulgare L.) cultivars, Sahara and Clipper, which differ in salinity tolerance. J. Exp. Bot. 60, 4089–4103. doi: 10.1093/jxb/erp243
Zeeshan, M., Lu, M., Sehar, S., Holford, P., and Wu, F. (2020). Comparison of biochemical, anatomical, morphological, and physiological responses to salinity stress in wheat and barley genotypes deferring in salinity tolerance. Agronomy 10:127.
Zeng, L., and Shannon, M. C. (2000). Salinity effects on seedling growth and yield components of rice. Crop Sci. 40, 996–1003.
Keywords: antioxidants, Cymbopogon, essential oil, geraniol dehydrogenase, medicinal and aromatic plant (MAP), oxidative stress, ROS, salinity
Citation: Mukarram M, Khan MMA, Zehra A, Petrik P and Kurjak D (2022) Suffer or Survive: Decoding Salt-Sensitivity of Lemongrass and Its Implication on Essential Oil Productivity. Front. Plant Sci. 13:903954. doi: 10.3389/fpls.2022.903954
Received: 24 March 2022; Accepted: 18 May 2022;
Published: 09 June 2022.
Edited by:
Loredana F. Ciarmiello, University of Campania Luigi Vanvitelli, ItalyReviewed by:
Jay Rangani, Central Salt and Marine Chemicals Research Institute, IndiaHeikham Evelin, Rajiv Gandhi University, India
Copyright © 2022 Mukarram, Khan, Zehra, Petrik and Kurjak. This is an open-access article distributed under the terms of the Creative Commons Attribution License (CC BY). The use, distribution or reproduction in other forums is permitted, provided the original author(s) and the copyright owner(s) are credited and that the original publication in this journal is cited, in accordance with accepted academic practice. No use, distribution or reproduction is permitted which does not comply with these terms.
*Correspondence: Mohammad Mukarram, mdmukarram007@gmail.com; mmukarram@myamu.ac.in; orcid.org/0000-0002-9034-9366