- 1Institute for Multidisciplinary Research in Applied Biology (IMAB), Universidad Pública de Navarra, Pamplona, Spain
- 2Group of Genetics, Breeding and Biochemistry of Brassicas, Mision Biologica de Galicia (MBG-CSIC), Pontevedra, Spain
- 3Laboratory of Fungal Genetics and Metabolism, Institute of Microbiology of the Czech Academy of Sciences, Prague, Czechia
- 4Microbiology and Genetics Department and Institute for Agribiotechnology Research (CIALE), University of Salamanca, Salamanca, Spain
Endophytic fungi of crops can promote plant growth through various mechanisms of action (i.e., improve nutrient uptake and nutrient use efficiency, and produce and modulate plant hormones). The genus Brassica includes important horticultural crops, which have been little studied in their interaction with endophytic fungi. Previously, four endophytic fungi were isolated from kale roots (Brassica oleracea var. acephala), with different benefits for their host, including plant growth promotion, cold tolerance, and induction of resistance to pathogens (Xanthomonas campestris) and pests (Mamestra brassicae). In the present work, the molecular and morphological identification of the four different isolates were carried out, describing them as the species Acrocalymma vagum, Setophoma terrestris, Fusarium oxysporum, and the new species Pyrenophora gallaeciana. In addition, using a representative crop of each Brassica U’s triangle species and various in vitro biochemical tests, the ability of these fungi to promote plant growth was described. In this sense, the four fungi used promoted the growth of B. rapa, B. napus, B. nigra, B. juncea, and B. carinata, possibly due to the production of auxins, siderophores, P solubilization or cellulase, xylanase or amylase activity. Finally, the differences in root colonization between the four endophytic fungi and two pathogens (Leptosphaeria maculans and Sclerotinia sclerotiorum) and the root glucosinolate profile were studied, at different times. In this way, how the presence of progoitrin in the roots reduces their colonization by endophytic and pathogenic fungi was determined, while the possible hydrolysis of sinigrin to fungicidal products controls the colonization of endophytic fungi, but not of pathogens.
Introduction
Endophytic fungi live asymptomatically inside plant tissues. In agriculture, endophytic fungi have been isolated from roots and aerial parts of several crops, mostly cereals (barley, maize, rice, sugarcane, and wheat) and soybean (Rana et al., 2019). These fungi have important benefits for their host plants by increasing tolerance to abiotic stresses (Khan et al., 2015) and resistance to biotic stresses, through direct action or by activating plant defensive responses (Poveda et al., 2020a; Poveda and Baptista, 2021). Furthermore, endophytic fungi promote plant growth by increasing access to nutrients (nitrogen, phosphorus, potassium, iron, zinc, etc.), production of plant hormones, reduction of the plant ethylene concentration, or an increased water acquisition rate (Poveda et al., 2021a). However, the use of endophytic fungi in a directed way in agriculture requires knowledge of their biological capacities and their behaviors in different crops and environments, the development of a large-scale production system, and their release to the market (Murphy et al., 2018). In that sense, in recent years numerous patents have been filed (i.e., 185 patents between 1988 and 2019 [Al-Ani, 2019; Ortega et al., 2020]), on the use of isolates from genera such as Neotyphodium, Muscodor, Curvularia, and Fusarium.
Plant species within the genus Brassica include important crops both agronomically and economically worldwide (Francisco et al., 2017). The U’s triangle classically explains the basic systematic relationship between the six major Brassica species (Nagaharu and Nagaharu, 1935). This system proposes that the three tetraploid species B. juncea (AABB genome), B. napus (AACC), and B. carinata (BBCC) are the derived allotetraploids of the diploid species B. rapa (AA), B. nigra (BB), and B. oleracea (CC), respectively, which arose by natural hybridization and chromosome doubling (Kim et al., 2018). Among the diploid species, kale (B. oleracea var. acephala) is a crop characterized by the formation of leaves along the stem, which are popular nowadays as a “superfood” as they contain phytochemicals beneficial to people’s health (Šamec et al., 2020).
Brassicas represent a unique case for us to study due to the production of glucosinolates (GSLs). GSLs are a class of sulfur-containing compounds found predominantly in the Brassicaceae plant family (Wu et al., 2021). These secondary metabolites are involved in numerous plant physiological processes, including adaptation to environmental conditions (Poveda et al., 2021b) and defense against pests and diseases (Eugui et al., 2022), including pathogenic fungi (Poveda et al., 2020b). Therefore, pathogenic fungi need to develop tolerance mechanisms to GSLs that infect Brassica tissues (Vela-Corcía et al., 2019). In this sense, endophytic fungi from horseradish (Armoracia rusticana) (Brassicaceae family) have been described as colonizing the roots when GSLs are used as a nutrient resource (Szűcs et al., 2018).
Conversely, few studies have focused on the diversity of endophytic fungi and their role as plant stimulators in Brassica crops (Card et al., 2015; Poveda et al., 2022). A previous study described, for the first time, the diversity of root endophytic fungi in kale roots, and how some isolates could promote plant growth and resilience (Poveda et al., 2020c).
Against that background, the objectives of this work were to study the biological properties of the four endophytic fungi widely isolated in kale and determine the role of GSLs in the colonization of these fungi using the Brassica U’s triangle.
Materials and methods
Biological material
The endophytic fungi used were isolated from B. oleracea var. acephala (kale) and identified by sequencing the internal transcribed spacer 1 (ITS1), 5.8S rDNA, and ITS2 as Fusarium sp. (MT628384), Pleosporales sp. A (MT628351), Pleosporales sp. B (MT628399), and Acrocalymma sp. (MT626728) (Vela-Corcía et al., 2019).
To test the role of GSLs in root colonization, two brassica root pathogenic fungi were used, Sclerotinia sclerotiorum and Leptosphaeria maculans. The S. sclerotiorum isolate MBG-Ss2 was originally isolated from naturally infected B. napus in an experimental field at MBG in January 2008 (Madloo et al., 2019). The L. maculans isolate CRD13/125/99 was provided by the Regional Diagnostic Center of the Regional Government of Castile and Leon (Salamanca, Spain), having been isolated from a B. napus field in Palencia (Spain) (Poveda, 2022).
The local kale (B. oleracea var. acephala) variety MBG-BRS0106 was utilized for in planta assays. Furthermore, for the growth promotion study of Brassica U’s triangle crops, B. oleracea (MBG-BRS0462), B. napus (MBG-BRS0063), B. rapa (MBG-BRS0163), B. juncea (EXT-BRS0184), B. nigra (EXT-BRS0185), and B. carinata (EXT-BRS0219) were used.
Taxonomic study of endophytic fungi
DNA extraction, amplification, and sequencing
Fungal DNA was extracted from mycelium scraped from a PDA culture using a commercial kit (Phire Plant Direct PCR Kit, Thermo Fisher Scientific). A ribosomal DNA region including the internal transcribed spacer 1 (ITS1), 5.8S rDNA, and ITS2 was amplified by PCR using primers ITS1 and ITS4 (White et al., 1990). The glyceraldehyde-3-phosphate dehydrogenase (GADPH) gene was amplified using the primer pair gpd-1 and gpd-2 (Berbee et al., 1999). The RNA polymerase II gene was amplified using RPB2-5 and RPB2-7c, and RPB2-7 and RPB2-11a (Liu et al., 1999). For large subunit rDNA (LSU), the LROR and LR5 primer pair was used (Vilgalys and Hester, 1990). Amplification conditions were 5 min at 98°C, 35 cycles at 98°C for 30 s, 54°C for 30 s, and 72°C for 60 s. PCR amplicons were purified following the manufacturer’s instructions (MSB Spin PCRapace, Stratec Biomedical, Germany) and sequenced through the Genomics Service at CACTI, University of Vigo, Spain.1 The GenBank accession numbers can be found in Supplementary Table S1.
DNA sequence analyses
The sequences obtained using the forward and reverse primers were assembled using the software package Seqman (v. 10.0.1) from DNAStar Inc. The resulting sequences were used for a BLAST search against the GenBank database. The datasets for phylogenetic analysis were obtained by downloading the sequences of accepted, closely related species from GenBank. Multiple sequence alignments were created in MAFFT v.7 (Katoh and Standley, 2013) using the G-INS-i method and edited in AliView v. 1.27 (Larsson, 2014). A suitable model of evolution for each alignment was selected in jModelTest v. 2.1.7 (Posada, 2008) using the BIC (Bayesian information criterion). The phylogenetic position of strains H22, H64, and H890 was demonstrated through maximum likelihood analysis of ITS (rDNA) in IQ-TREE v. 2.1.2 (Minh et al., 2020). The statistical support was determined by 1,000 standard bootstrap replicates. In the case of isolate H441, which is described as a new species in this study, single-locus maximum likelihood analysis was performed in the same way as mentioned above using DNA sequences of four loci, ITS (rDNA), LSU (rDNA), GAPDH, and RPB2. Furthermore, concatenated phylogenetic trees were calculated using partitioned analysis IQ-TREE v. 2.1.2 based on sequences of all four loci. The resulting phylogenetic trees were prepared in iTOL (Interactive Tree Of Life) (Letunic and Bork, 2021). Only significant bootstrap values (higher than 70) are presented in the final phylograms.
Fungal morphological study
Fungi were cultivated on potato dextrose agar (PDA) (Sigma-Aldrich, St. Louis, United States) at 25°C in a 12 h day/night cycle for 5 days. Morphologies of fungi structures were investigated using a Leica DM4500 microscope (Leica, Germany) equipped with a Retiga 2000R camera (QUIMAGING, Canada). At least 24 measurements per structure were taken.
In vitro plant growth promoting assays
Solubilization of insoluble phosphate (CaH2PO4) and potassium (AlKO6Si2) was performed using YED-P and Aleksandrov media, respectively (Hu et al., 2006; Jiménez-Gómez et al., 2020). If a clear halo around the mycelium appeared within 7 days of incubation at 28°C, it was considered a positive result. Auxins and siderophore production were analyzed by the colorimetric method (Khalid et al., 2004) and in M9-CAS-agar medium (Jiménez-Gómez et al., 2019), respectively. The production of siderophores was analyzed qualitatively, by means of the production of specific yellow halos. For the quantification of auxins produced, a standard line was made with the 3-indoleacetic acid (IAA) standard (I3750, Sigma-Aldrich, Missouri United States) by spectrophotometry at 530 nm.
Enzymatic activity assays
To detect cellulolytic, xylanolytic, or amylolytic activities, TSA medium was supplemented with 1% CMC (carboxymethyl cellulose), xylan, or starch, respectively (Garcia-Fraile et al., 2007). Briefly, a mycelium plug of each fungal isolate was placed on a different screening plate, which were incubated at 24°C for 7 days. Appearance of halos, which were observed after staining, indicated the degradation of the substrate. Cellulose- and xylan-supplemented plates were soaked in Congo Red and clarified with 1 M NaCl solution (Mateos et al., 1992). Starch-supplemented plates were soaked with lugol solution (potassium iodine, Panreac®) for a few seconds, until a halo appeared (Petzel and Hartman, 1986).
In planta growth promotion In Brassica U’s triangle crops
For the in planta assays, a representative of each of the vertices and edges of the Brassica U’s triangle was used: B. napus, B. rapa, B. oleracea var. acephala, B. juncea, B. nigra, and B. carinata. The plants were grown in 10 l pots with sterile (80°C, 24 h) peat substrate (Profi-Substract, Gramoflor, Valencia, Spain) and their roots inoculated with each endophytic fungus. Twelve plants were inoculated per fungus, leaving 12 plants uninoculated as a control. Fungal inoculation was performed as described by Poveda et al. (2019) for B. napus. At 4 weeks post-fungi inoculation, fresh and dry (48 h at 60°C) weights were taken from the aerial parts of the plants.
Root colonization assays with endophytic and pathogenic fungi
Following the methodology described in the previous section, kale plants were inoculated with the four endophytic fungi and two pathogenic fungi, to analyze colonization differences related to the profile of root GSLs for different modes of interaction with Brassica plants. The plants were grown in 3 l pots with a substrate consisting of peat moss (Profi-Substract, Gramoflor, Valencia, Spain) previously treated at 80°C for 24 h, and their roots were inoculated with each fungus. Thirty 3-week-old plants were inoculated per fungus with 1 ml of a conidial suspension at 2 × 107 spore ml−1 (determined using a hemocytometer), keeping a further 30 plants free of inoculation as controls. For S. sclerotiorum, all the mycelium formed in three PDA Petri dishes was collected and mixed with 30 ml of sterile distilled water in a Falcon tube. Subsequently, 1 g of Ballotini glass balls (0.15–0.25 mm and 1 mm diameter; 0.5 + 0.5 g, respectively) (Potters, Saint-Pourçain-sur-Sioule, France) was added, vigorously shaking for 20 min. A mycelium suspension was obtained, which was adjusted to the absorbance of 0.17 per mL at 520 nm, for inoculation.
Subsequently, the roots of nine plants per fungal inoculation were collected and washed with water (freezing them directly in liquid nitrogen; three pools, each representing all the roots of three different plant), at different post-fungi-inoculation times: 7, 12, and 17 days. The different root pools were used to quantify the fungal root colonization by qPCR and GSL profiles.
Quantification of fungal root colonization
Quantification of fungal DNA in kale roots was performed by qPCR as previously described by Velasco et al. (2021), with some modifications. DNA was extracted from roots of the untreated (control) and fungal (endophytes and pathogens)-inoculated plants, using the methodology previously described in this work (section 2.2.1). A mix was prepared in a 20 μl volume using 100 ng of DNA, 10 μl of Brilliant SYBR Green QPCR Master Mix (Roche, Penzberg, Germany), the forward and reverse primers at a final concentration of 600 nM, and nuclease-free PCR-grade water (to adjust the final volume). Different endogenous genes of each of the fungi and kale were used (Supplementary Table S2). Amplifications were performed in a 7,500 Real-Time PCR System (Applied Biosystem, Forster City, CA, United States) programmed for 40 cycles under the following conditions: denaturation, 95°C for 15 s; annealing, 60°C for 1 min; extension, 72°C for 1 min. DNA extracted from the root pools of each fungal inoculation and for each post-inoculation time was used, performing each PCR in triplicate. Cycle threshold values assisted us to calculate the amount of fungal DNA using standard curves. The values obtained for fungus DNA were referenced against the values obtained for kale DNA in each of the samples.
GSLs analysis
Analysis of the GSL profiles in the samples was carried out following the methodology described by Kliebenstein et al. (2001), with minor modifications. Twelve milligrams of freeze-dried kale root powder was mixed with 400 μl of 70% (v/v) methanol preheated to 70°C, 10 μl of PbAc (0.3 M) and 120 μl of ultra-pure water preheated to 70°C, and 20 μl of glucotropaeolin (added as the internal standard). After shaking in a microplate incubator (OVAN Orbital Midi; OVAN, Badalona, Spain), 400 μl of the glucosinolate extracts were pipetted into an ion-exchange column with Sephadex DEAE-A25 (Sigma-Aldrich, St. Louis, MO, United States). For desulfation, purified sulfatase (E.C. 3.1.6.1, type H-1 from Helix pomatia; Sigma-Aldrich, St. Louis, MO, United States) was added. Finally, the desulfated GSLs were diluted in 200 μl of ultra-pure water and 200 μl of 70% methanol and kept frozen for further analyses.
The chromatographic analyses were conducted on a UHPLC (Nexera LC-30 AD; Shimadzu, Kyoto, Japan) equipped with an injector (Nexera SIL-30 AC, Shimadzu, Kyoto, Japan) and one SPDM20A UV/VIS photodiode array detector (Shimadzu, Kyoto, Japan). UHPLC column X Select ®HSS T3 (2.5 μm, 2.1 × 100 mm i.d.) from Waters (Waters Corporation, Milford, MA, United States) was used, which was protected with a VanGuard pre-column. GSLs were separated in aqueous acetonitrile, with a flow of 0.5 ml min− 1:1.5 at 100% H2O, an 11 min gradient from 5 to 25% (v/v) acetonitrile, 1.5 min at 25% (v / v) acetonitrile, a minute gradient from 25 to 0% (v/v) acetonitrile, and a final 3 min at 100% H2O; then, they were quantified at 229 nm. Identification of the GSLs was carried out by comparison with commercial standards (Phytoplan, Heidelberg, Germany).
Statistical analysis
To compare the means for each fungal inoculation treatment with the control, Student’s t-test was used at p < 0.05 for each assay, using the measurements of 10 plants per treatment for plant growth quantification and the measurements of three root pools with three complete roots each for GSLs analysis. To quantify the colonization, one-way ANOVA using Tukey’s multiple range test was used, by analyzing the measurements of three root pools with three complete roots each.
Results
Phylogenetic analysis
To identify the four isolates, several phylogenetic trees were created. Identification of the fungal strains isolated in this study was carried out through a BLAST search against the GenBank database using ITS sequences, which gave the following results: strain H22 (formerly named: Acrocalymma sp.; Poveda et al., 2020a) is a representative of Acrocalymma vagum, H64 (formerly named: Pleosporales sp. A; Poveda et al., 2020a) of Setophoma terrestris, and H890 (formerly named: Fusarium sp.; Poveda et al., 2020a) of Fusarium oxysporum. The results of our maximum likelihood analysis representing the phylogenetic relationships with closely related species are depicted in Figure 1 and the fungal morphology in Supplementary Figures S1–S3. On the other hand, the BLAST search for strain H441 (formerly named: Pleosporales sp. B) failed to identify the strain to the species level. Based on the results of a phylogenetic analysis using the four DNA loci depicted in Figure 2 and Supplementary Figure S4, strain H441 was described as the new species Pyrenophora gallaeciana sp. nov., forming a clade with P. nobleae, P. fugax, P. novozelandica, and P. phaeocomes in single-locus trees based on ITS and GAPDH, and clustering with P. chaetomioides and P. lolii in an RPB2 tree (albeit with very low support). The tree based on LSU sequences was poorly resolved because of little variation in the alignment. In the tree calculated from all four loci, P. gallaeciana was located in the clade with P. nobleae, P. fugax, P. novozelandica, and P. phaeocomes. This clade, as well as other deep nodes, acquired quite high bootstrap support, unlike trees based on single-locus alignment, but this is likely an artifact caused by concatenation (Kubatko and Degnan, 2007).
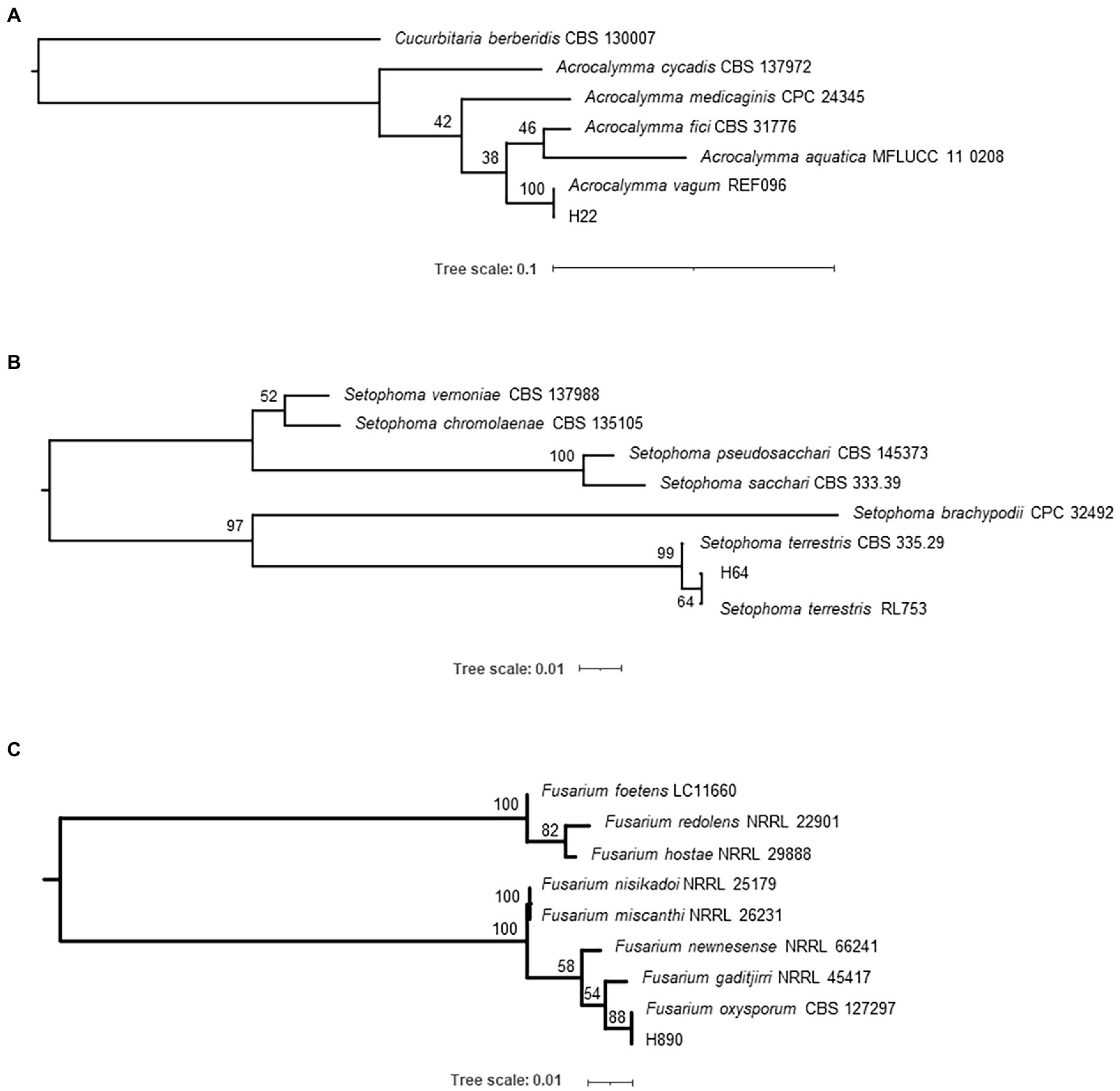
Figure 1. Phylogenetic trees displaying the relationships between isolates H22 (A), H64 (B), and H890 (C). The trees were calculated in IQ-TREE 2.1.2 using ITS (rDNA) sequences with a TrNef+G substitution model and 1,000 standard bootstrap repeats.
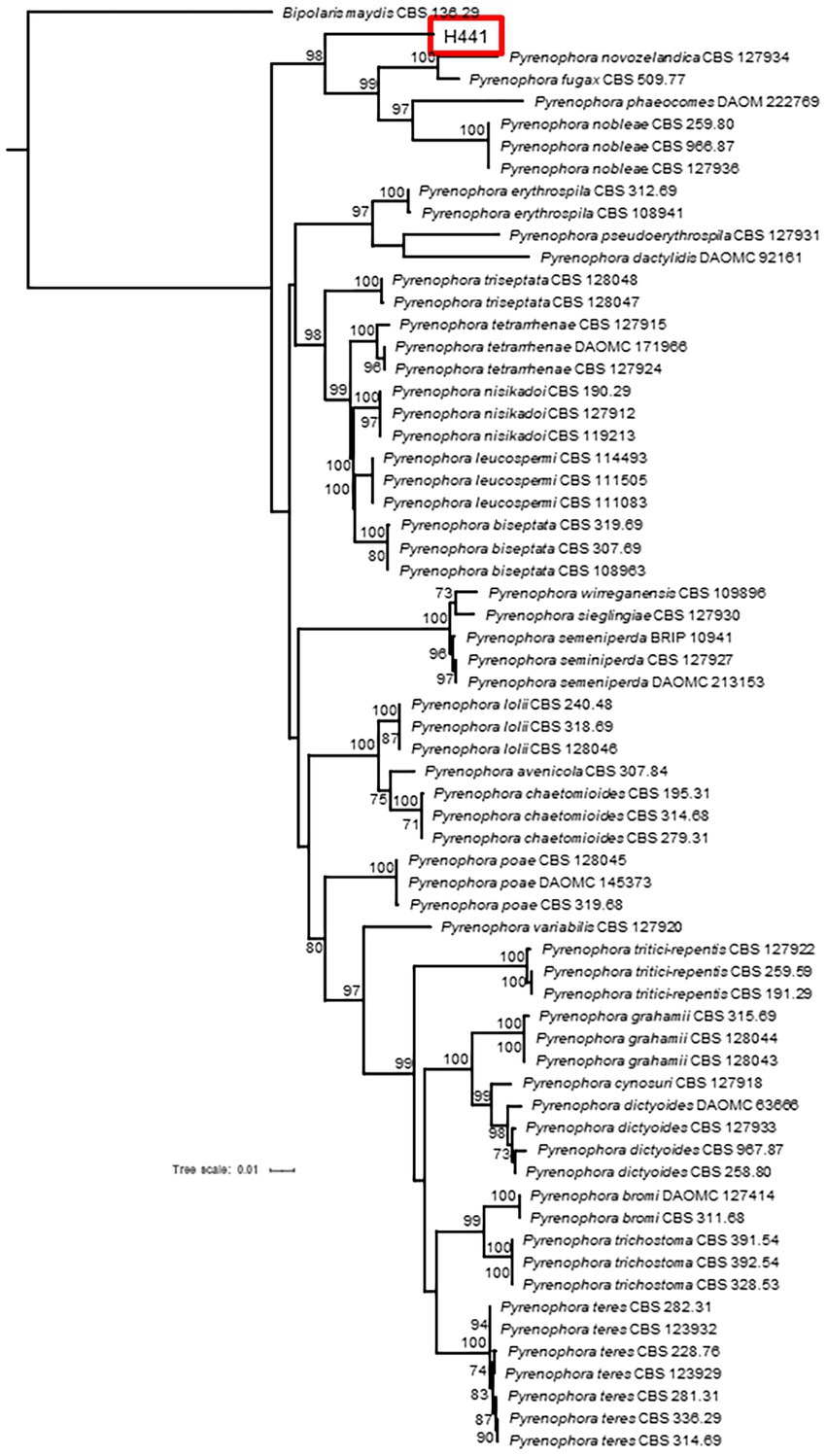
Figure 2. Phylogenetic tree displaying the relationship of isolate H441 with several species of genus Pyrenophora. The tree was calculated by partitioned analysis in IQ-TREE 2.1.2 using DNA sequences from four loci (GAPDH—HKY + I + G; ITS—TrNef+I + G; LSU—K80 + I; RPB2—K80 + I + G) and 1,000 standard bootstrap repeats.
Pyrenophora gallaeciana J. Poveda, V. M. Rodríguez and P. Velasco, sp. nov. MycoBank MB- 842942. Figure 3 .
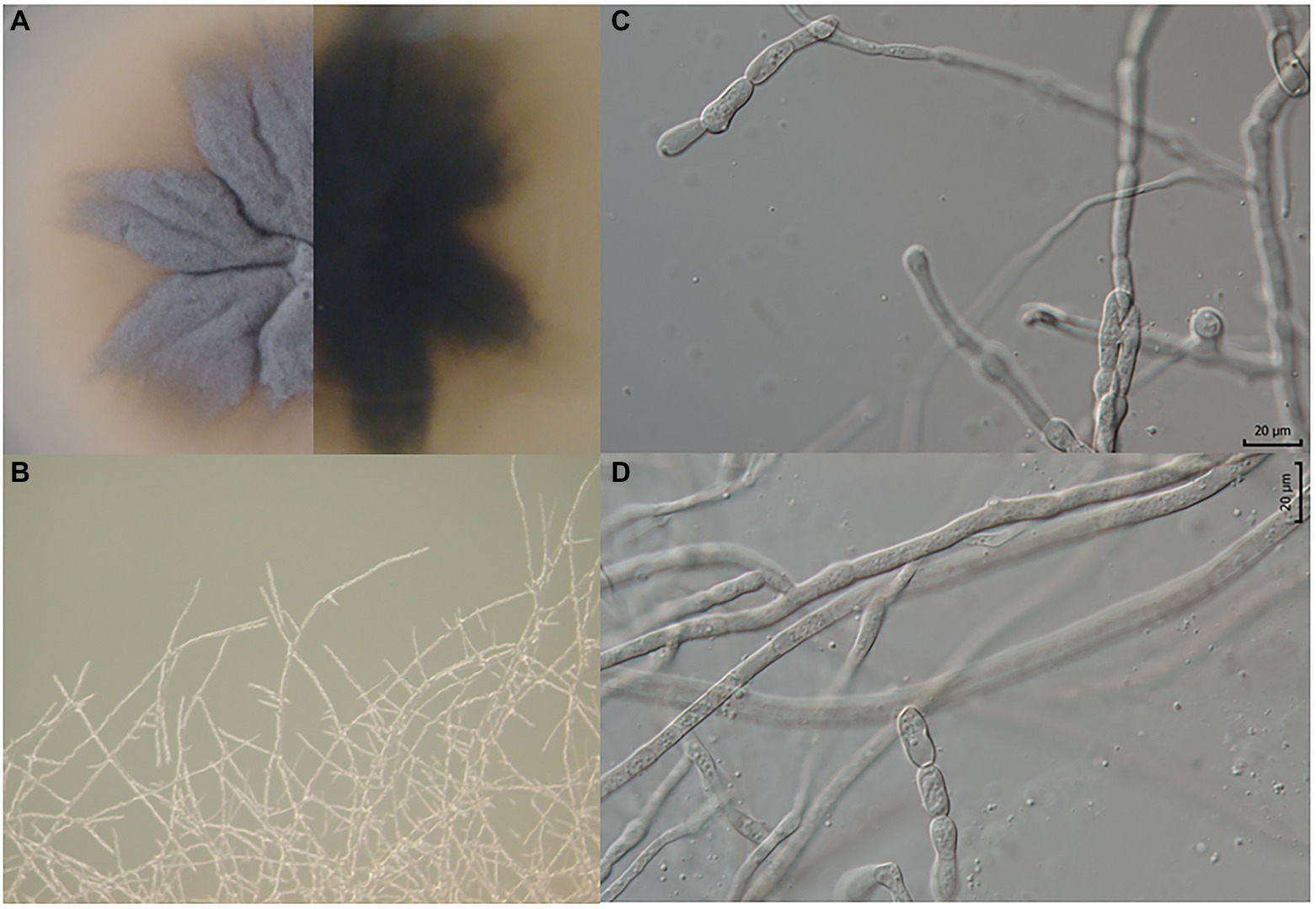
Figure 3. Pyrenophora gallaeciana. (A) Colony on PDA (front and reverse) (120 h old); (B) mycelium on PDA (120 h old); (C, D) structures probably involved in asexual reproduction.
Etymology: gallaeciana [gal·lækiana]: pertaining to Gallaecia (Galicia), the northwestern region of Spain, where the representatives of this species were isolated.
Typus: Spain, Pontevedra, Misión Biológica de Galicia, from roots of B. oleracea var. acephala, May 2016, J. Poveda, V. M. Rodríguez, and P. Velasco (holotype: CECT-21208, culture ex-type CECT-21208 = CCF 6607 = MB842942).
Colony diam, 25°C, 7 d (mm): PDA: 45.3.
Culture characteristics, 25°C, 7 d: PDA: The isolate produced dim gray (6D6C72) upper and raisin black (27292B) bottom colonies. The colony morphology is characterized by irregular margins, a wrinkled form, and crateriform elevation (Figure 3A).
Micromorphology: Branched hyphae 2.5 μm thick (min.: 2 μm; max.: 3.5 μm) (Figures 3B,C). Constricted hyphae, structures (25–35 × 6–10 μm) probably involved in asexual reproduction, possibly conidia, but with different (much simpler) morphology in comparison to other Pyrenophora species (Figures 3C,D).
Analysis of PGP and enzymatic traits
The potential of the endophytic fungi to promote plant growth and their ability to hydrolyze plant cell compounds (first step to enter the plant tissues) were analyzed through in vitro tests. The results are summarized in Table 1. The four endophytic fungal isolates could produce IAA-like molecules at different levels. A. vagum did not demonstrate any other PGP mechanism. S. terrestris had the ability to solubilize phosphorus and engage in xylanase and amylase activity. P. gallaeciana and F. oxysporum could produce siderophores and, also, engaged in cellulase and xylanase activity. Furthermore, P. gallaeciana exhibited amylase activity.
Growth promotion In Brassica U’s triangle crops
The four endophytic fungi under study were used to evaluate the growth of the six Brassica species that make up the U’s triangle. Root inoculation with the endophytic fungus A. vagum caused an increase in the weight of the aerial part of B. juncea and B. nigra compared to uninoculated plants, and significantly reduced the fresh weight of B. carinata. In the case of S. terrestris, a significant increase in fresh weight was reported in B. nigra and B. carinata compared to uninoculated plants, and a significant reduction in B. napus. The presence of P. gallaeciana in roots of B. rapa, B. juncea, and B. nigra significantly increased the fresh weight of the aerial part compared to the plants without fungal inoculation. Finally, root inoculation with F. oxysporum produced an increase in the fresh weights of B. rapa, B. juncea, and B. carinata compared to uninoculated plants (Figure 4). The same biomass trend was reported by analysing dry weight (Supplementary Figure S6).
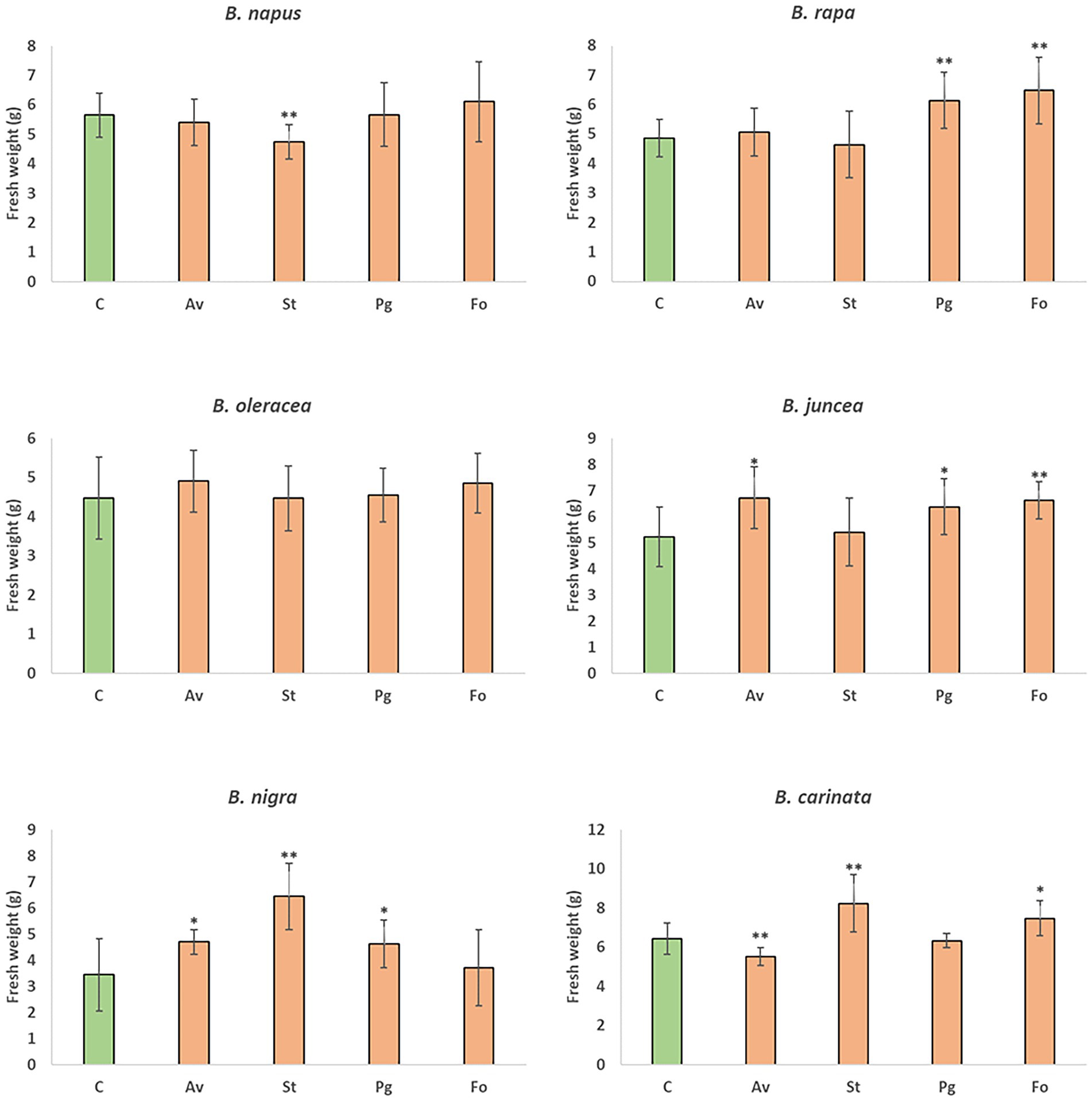
Figure 4. Mean of fresh weights of B. napus, B. rapa, B. oleracea var. acephala, B. juncea, B. nigra, and B. carinata plants grown in greenhouse. Plants without inoculation (C) and those inoculated with A. vagum (Av), S. terrestris (St), P. gallaeciana (Pg), and F. oxysporum (Fo) were gathered at 12 weeks old. The fresh weights of the aerial parts of 10 plants for treatment were measured and are shown as the mean ± standard deviation. Differences between treatments were calculated using Student’s t-test. Asterisks denote significant differences at p ≤ 0.05 (*) and p ≤ 0.01 (**).
Root colonization assays with endophytic and pathogenic fungi
To study the role of GSLs in the colonization of Brassica roots by pathogenic and endophytic fungi, kale plants were inoculated with the four endophytic fungal isolates (A. vagum, S. terrestris, P. gallaeciana, and F. oxysporum) and two pathogens (L. maculans and S. sclerotiorum) separately.
In the roots collected 7 days post-inoculation, F. oxysporum fungus colonized the roots least significantly. Meanwhile, A. vagum and S. terrestris colonized kale roots significantly more (Figure 5). Both fungi continued to colonize the roots with the greatest significance 12 days post-inoculation, followed in order of significance by L. maculans, P. gallaeciana, and S. sclerotiorum. The fungus that colonized kale roots to a lesser extent continued to be F. oxysporum (Figure 5). Finally, 17 days post-inoculation, the four endophytic fungi (A. vagum, S. terrestris, P. gallaeciana, and F. oxysporum) colonized the roots without significant differences between them. The two pathogenic fungi (L. maculans and S. sclerotiorum) showed significantly higher kale root colonization (Figure 5).
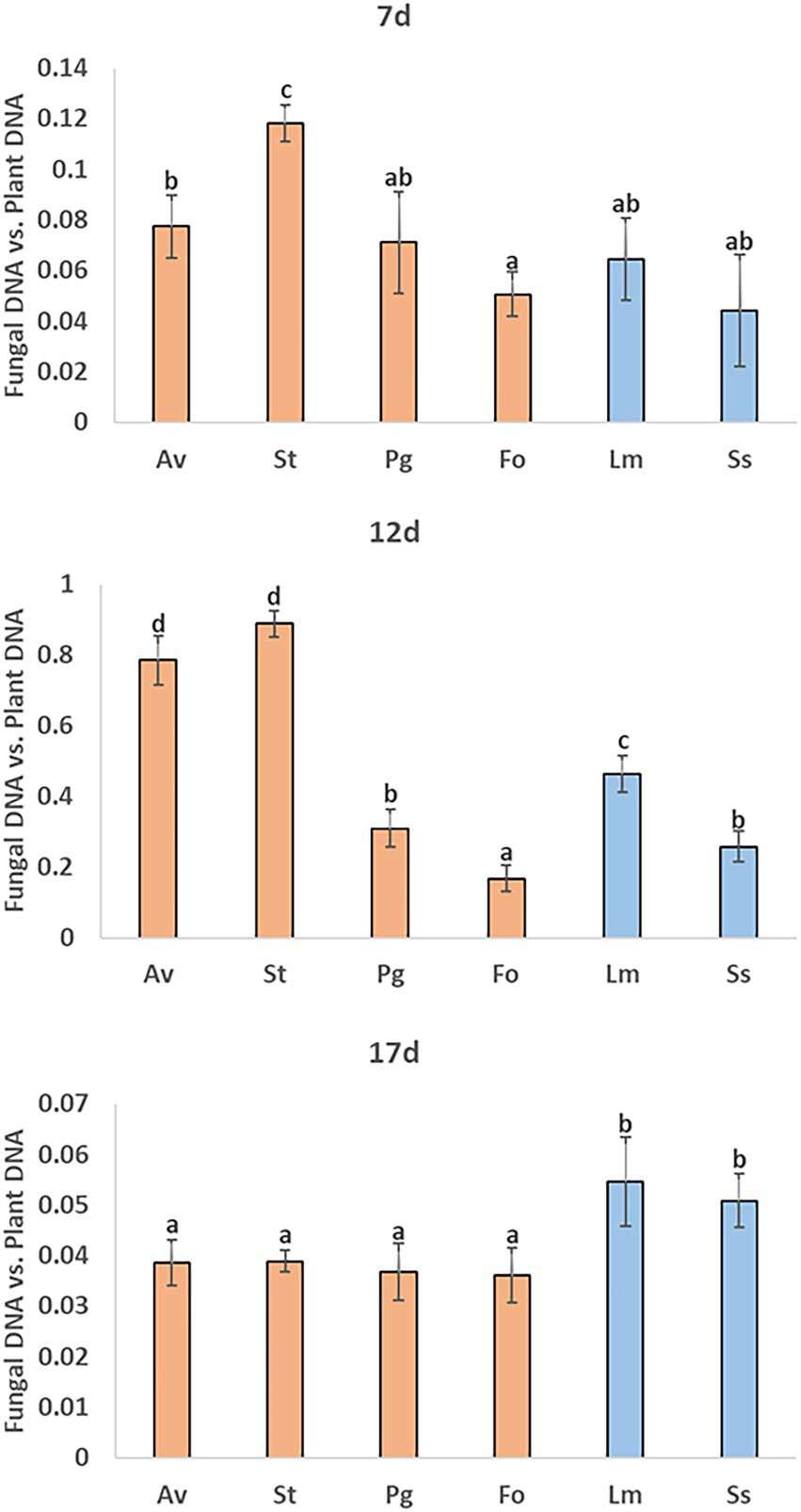
Figure 5. Measurements of kale root colonization by different endophytic and pathogenic fungi using qPCR. A. vagum (Av), S. terrestris (St), P. gallaeciana (Pg), and F. oxysporum (Fo) inoculated roots were collected 7-, 12-, and 17-days post-inoculation. Values are the proportion of fungal DNA to plant DNA; means of three root pools (with three plants each) are given with the corresponding standard deviations. One-way analysis of variance (ANOVA) was performed, followed by the Tukey’s test. Different letters represent significant differences (p < 0.05).
GSL profiles
At early stages of inoculation (7 days post-inoculation), fungus colonization significantly reduced the levels of root GSLs. This reduction affected aliphatic and indolic GSLs equally in all tested fungi (Figure 6). However, at 12 days post-inoculation, plants inoculated with endophytic fungi recovered the control amount of GSLs, mainly due to a higher quantity of indolic GSLs, since aliphatic GSLs remained at significantly lower levels that those observed with the control. Roots inoculated with pathogenic fungi accumulated higher levels of total GSLs; there was no common pattern between the two fungi since this increase in plants inoculated with S. sclerotiorum was due to the accumulation of aliphatic GSLs, whereas plants inoculated with L. maculans accumulated significantly more indolic GSLs. Surprisingly, at 17 days post-inoculation, a significant decrease in the total GSL content was observed, except for in plants inoculated with S. sclerotiorum. Quantified aliphatic GSLs included progoitrin, sinigrin, and glucoiberverin. In the case of progoitrin, a significant decrease in its 7 day post-inoculation content was quantified in roots inoculated with A. vagum, S. terrestris, L. maculans, or S. sclerotiorum, in comparison with uninoculated plants. The results 12 days post-inoculation continued to be significantly lower in progoitrin in inoculations with A. vagum, S. terrestris, or L. maculans. Meanwhile, 17 days post-inoculation, only the roots inoculated with L. maculans showed significant lower levels compared to uninoculated plants (Figure 6). With respect to sinigrin, root inoculation with any fungus caused a significant decrease in kale roots’ sinigrin content compared to uninoculated plants 7 days post-inoculation. The same significantly lower levels were quantified 12 days post-inoculation and 17 days post-inoculation with all fungi, except for S. sclerotiorum (at both times) and L. maculans (at 17 days post-inoculation) (Figure 6). Finally, in the case of glucoiberverin, in the roots inoculated with fungi S. terrestris, P. gallaeciana, F. oxysporum, or L. maculans had significantly lower content glucoiberverin contents 7 days post-inoculation compared to uninoculated plants. Then, 12 days post-inoculation, roots inoculated with A. vagum, S. terrestris, P. gallaeciana or L. maculans had significantly lower levels of glucoiberverin than uninoculated plants. However, roots inoculated with S. sclerotiorum had significantly higher GSL levels. These significantly higher levels were also quantified in S. sclerotiorum 17-days-post-inoculation. In the cases of S. terrestris and L. maculans, significantly lower levels were maintained than in the uninoculated plants (Figure 6).
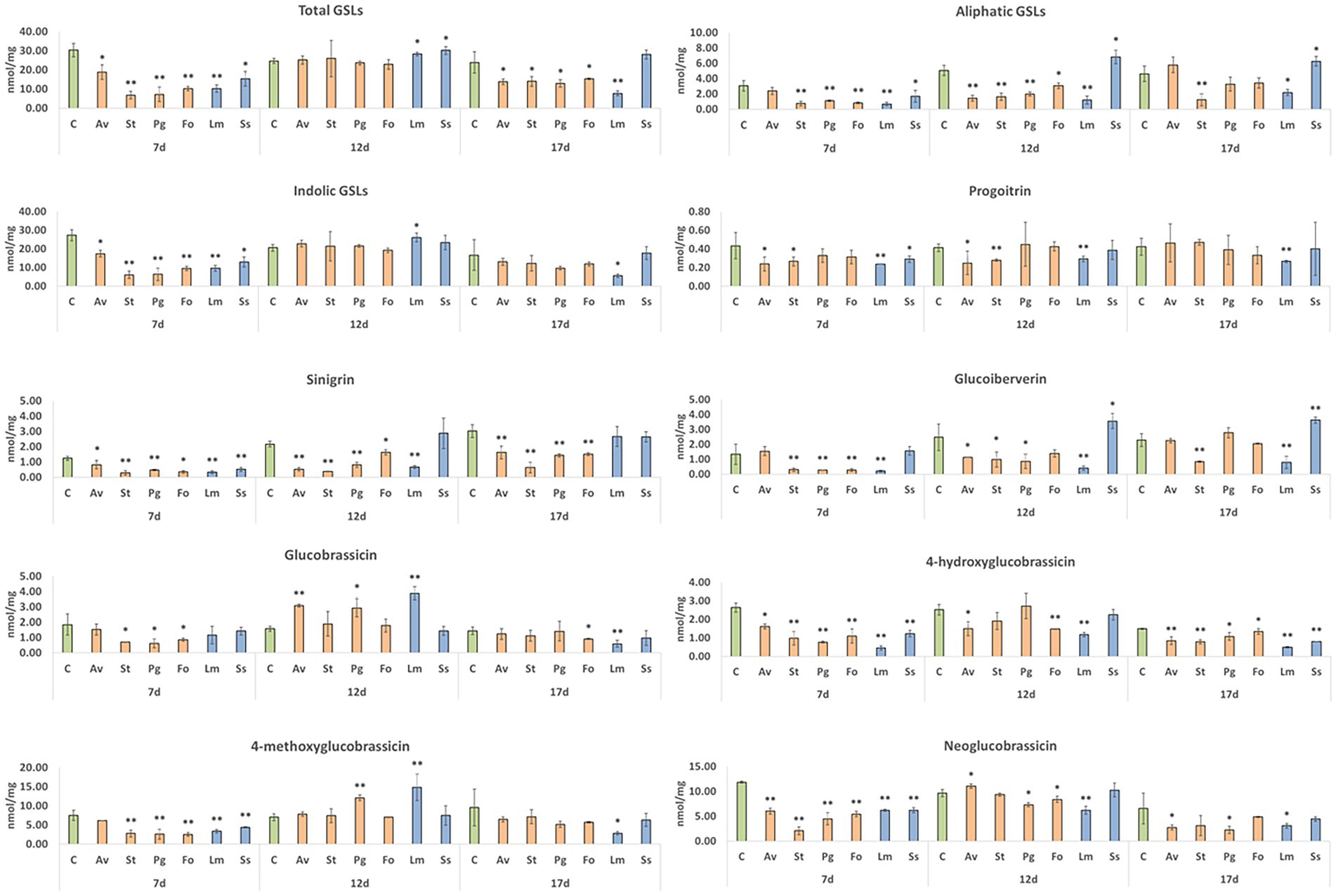
Figure 6. GSL content in kale roots colonized by different endophytic and pathogenic fungi. A. vagum (Av), S. terrestris (St), P. gallaeciana (Pg), and F. oxysporum (Fo) inoculated roots were collected 7-, 12-, and 17-days post-inoculation. Data are the means of three root pools (with three plants each) per inoculation with the corresponding standard deviations. Student’s t-test was performed. Asterisks denote significant differences at p ≤ 0.05 (*) and p ≤ 0.01 (**).
As far as indolic GSLs are concerned, glucobrassicin, 4-hydroxyglucobrassicin, 4-methoxyglucobrassicin, and neoglucobrassicin were quantified. The glucobrassicin content decreased significantly in roots inoculated with S. terrestris, P. gallaeciana, or F. oxysporum compared to the uninoculated plants at 7 days post-inoculation. However, 12 days post-inoculation, a significant increase was reported in roots inoculated with A. vagum, P. gallaeciana, or L. maculans. Seventeen days post-inoculation, a significant reduction in the root contents of glucobrassicin was quantified with the fungi F. oxysporum and L. maculans (Figure 6). In the case of 4-hydroxyglucobrassicin, both 7 and 17 days post-inoculation, the root contents were significantly reduced with the inoculation of all fungi compared to the uninoculated plants. However, 12 days post-inoculation, only the 4-hydroxyglucobrassicin content was significantly reduced with the fungi A. vagum, F. oxysporum, and L. maculans (Figure 6). Seven days post-inoculation, a reduction in the root 4-methoxyglucobrassicin contents of kale plants was quantified with all fungal inoculations, except for A. vagum, compared to uninoculated plants. In contrast, 12 days post-inoculation, there was a significant increase in roots inoculated with P. gallaeciana and L. maculans compared to uninoculated plants. At 17 days post-inoculation, a significant reduction in root inoculation with L. maculans was only found (Figure 6). Finally, in the case of neoglucobrassicin, significantly lower levels were reported in roots inoculated with all fungi compared to uninoculated plants at 7 days post-inoculation. However, 12 days post-inoculation, root inoculated with A. vagum, P. gallaeciana, F. oxysporum, or L. maculans showed significantly higher neoglucobrassicin contents than uninoculated plants. Finally, the plants inoculated with A. vagum, P. gallaeciana, or L. maculans demonstrated significantly lower levels compared to uninoculated plants at 17 days post-inoculation (Figure 6).
Discussion
Endophytic fungi have received increasing interest in recent years. However, the numbers of isolates and newly described fungi are still low, especially within Brassica crops (Poveda et al., 2022). The ability of different endophytic fungi to promote plant growth in crops, thanks to various mechanisms, has been widely reviewed in recent years (Rana et al., 2019; Rigobelo and Baron, 2021). Although some microbes are adapted to an endophytic lifestyle, to reach roots’ inner tissues, they must first compete and succeed in the rhizosphere, where they can benefit the plant health by providing nutrients and hormones (Jiménez-Gómez et al., 2020). It is important to note that the plant growth-promoting ability may be fungal strain- and plant genotype-specific (Rigobelo and Baron, 2021; Poveda et al., 2021a).
In this study, the specific taxonomic classification of four species of endophytic fungi from kale (B. oleracea var. acephala) was carried out, with one of them described as a new species. These fungal isolates were obtained in a previous work, although only identified to order or genus level (Poveda et al., 2020a). The four species of endophytic fungi taxonomically described in this work represent the first description, at the species level, of endophytic fungi from kale. These results highlight the importance of studying the diversity of endophytic fungi present in Brassica crops.
Here, the production of auxins at different levels was a common PGP trait among the isolates. Although it is known that some strains of A. vagum can increase auxin synthesis in plant hosts (Liu and Wei, 2019), this was the first description of the fungus as a putative producer of IAA-like compounds. This mechanism could be involved in its ability to promote the growth of the Brassica U triangle crops B. juncea and B. nigra, the growth promotion previously reported in kale (Poveda et al., 2021a), or Medicago sativa growth (Hou et al., 2019). However, in this work the root inoculation of B. carinata with A. vagum causes a reduction in plant growth, an aspect not previously mentioned for any crop.
The ability of F. oxysporum to produce auxins has been previously described, along with its role in promoting the growth of maize (Mehmood et al., 2018). However, our F. oxysporum isolate represents the first description of an endophytic isolate of this species as a producer of siderophores. Cellulolytic and xylanase activity have been previously reported for endophyte F. oxysporum (Onofre et al., 2013; Farouk et al., 2020). All of these mechanisms could be involved in the growth promotion reported in B. juncea, B. rapa, and B. carinata, as was previously verified with kale (Poveda et al., 2021a).
The S. terrestris isolate solubilizes phosphorus, and it carries out xylanase and amylase activity; ours is the first description of a member of the genus Setophoma showing these capacities. Although Setophoma species have been previously described as endophytes of Brassicaceae plants, such as horseradish (Armoracia rusticana; Szűcs et al., 2018) or kale (Poveda et al., 2021a), this study presents the first description of plant growth-promotion activity by a Setophoma species, particularly in B. nigra and B. carinata. However, our results showed growth reduction when B. napus roots were inoculated. A previous study reported the first case of pink root rot in B. napus produced by S. terrestris (Yang et al., 2017). The thin line between beneficial and pathogenic behavior of some endophytic fungi, when interacting with different hosts, needs further research.
In this work, a new species of endophytic fungi is described: P. gallaeciana (meaning: from Galicia, Spain). P. gallaeciana-type isolates could produce auxins and siderophores and carry out cellulase, xylanase, and amylase activities. Members of the genus Pyrenophora, such as the barley fungal pathogens P. teres f. sp. teres and P. graminea (Ellwood et al., 2010; Bakri et al., 2011; Ismail et al., 2014), showed similar mechanisms. Here, P. gallaeciana is a plant growth promoter in B. juncea, B. rapa, and B. nigra, which is consistent with previous results on the Pyrenophora-kale interaction (Poveda et al., 2021a).
The PGP results obtained with these fungal isolates also report important differences according to the mode of application. Poveda et al. (2020a) obtained a higher kale plants growth with inoculation with beet pulp colonized by F. oxysporum or P. gallaeciana (then identified as Fusarium sp. and Pleosporales sp. B, respectively). However, in our current work, inoculation with spores of both fungi did not report a PGP. It is noteworthy that the application of spores allows knowing the exact amount of inoculated endophyte fungus, while the use of mycelium colonizing beet pulp does not, making it impossible to make a real comparison between different fungi. These differences were also reported with the isolate Trichoderma hamatum, which did not report PGP capacity in kale plants in its application as mycelium in beet pulp (Poveda et al., 2020c), but showed PGP when applied as spores (Velasco et al., 2021).
The Brassicaceae plants’ pathogenic fungi need to tolerate or eliminate GSLs from plant tissues to colonize and infect them. Botrytis cinerea tolerates GSLs through the mfsG membrane transporter, which rapidly removes the products of GSLs’ hydrolysis (Vela-Corcía et al., 2019). S. sclerotiorum acts directly on these toxic hydrolysis products through an isothiocyanate hydrolase (Chen et al., 2020). In the specific case of endophytic fungi, GSLs’ tolerance that supports them to colonize their hosts’ tissues is not fully understood (Poveda et al., 2022). In the case of Trichoderma, the production of myrosinase-binding proteins modifies the hydrolysis of GSLs to allow/contribute to Brassicaceae root colonization (Poveda et al, 2019), with the indole GSLs involved in controling the level of colonization (Poveda, 2021). Other endophytic fungi, including F. oxysporum and S. terrestris, directly degrade the GSLs present in their hosts and use them as nutrients (Szűcs et al., 2018).
Brassicas are non-mycorrhizal plants, with the presence of GSLs precisely proposed as the reason why these plants cannot be mycorrhized (Vierheilig et al., 2000; Anthony et al., 2020). These plants have been described as the main metabolites involved in the control of the endophytic fungal microbiota on this plant family (Plaszkó et al., 2022). Therefore, root colonization by endophytic fungi in Brassica plants must be closely related to the GSL content and profile of plant tissues.
The analyses of root colonization levels at 12 days post-inoculation revealed no differences between mock-inoculated plants and endophytic fungi-inoculated ones. Conversely, pathogenic fungi-inoculated plants showed an increased level of colonization. Specifically, with S. sclerotiorum, aliphatic GSLs increased. This was previously described in A. thaliana infection, where S. sclerotiorum induces the expression of synthesis genes (Stotz et al., 2011). Meanwhile, with L. maculans, indole GSLs increased, something previously observed in B. rapa (Abdel-Farid et al., 2010).
Two common aspects were found as corelated in all endophytic fungal strains vs. tested pathogenic fungal strains at 17 days post-inoculation: root colonization and the sinigrin content. The sinigrin contents were similar for root colonization with any pathogenic strain. However, the sinigrin contents decreased with endophytes’ root colonization. This is possibly due to sinigrin hydrolysis by endophytic fungi and further fungicide activity of the hydrolysis residuals, such as allyl isothiocyanate (Vandicke et al., 2020). Our results suggest that low sinigrin levels detected in roots inoculated with isolates from P. gallaeciana and F. oxysporum are directly related to their lower levels of root colonization. However, P. gallaeciana kale roots’ colonization reached its maximum at 12 days post-inoculation compared to roots inoculated with F. oxysporum. In both cases, the 4-hydroxyglucobrassicin content was lower than in mock-inoculated plants, except for roots inoculated with P. gallaeciana at 12 days post-inoculation. Thus, 4-hydroxyglucobrassicin hydrolysis products may inhibit both fungi’s root colonization, at different timepoints depending on the fungal strain.
Kale root colonization by endophytes A. vagum and S. terrestris was higher than for the other fungi at 7 and 12 days post-inoculation, possibly due to a decrease in progroitrin levels, which does not occur 17 days post-inoculation. Similarly, increased root colonization by L. maculans at 12 days post-inoculation could also be related to a decrease in the root progoitrin content. Similar GSL profiles for roots colonized by fungal endophytes and certain fungal pathogens could be related to the hemibiotrophic lifestyle, which involves an early stage of endophytism not present in the necrotrophic lifestyle (Newton et al., 2010). Accordingly, lower levels of progoitrin may be related to greater plant colonization by fungal endophytes and pathogens.
Endophytic fungi could induce the expression of synthesis genes, the activation of plant enzymes that hydrolyze them, or act directly against them (Poveda et al., 2022). In this sense, differences in tolerance and/or degradation of GSLs by endophytic and pathogenic fungi may be explained by specific genomic comparisons. However, the sequences of these species are not yet available. Furthermore, the GSL content in the roots does not depend on localized synthesis but can be transported from other plant organs such as leaves (Witzel et al., 2015). Although our results provide some insights, the mechanisms involved in the changes in GSL levels in Brassica roots require further research.
In conclusion, this work presents not only the first description at the species level of endophytic fungi of a B. oleracea crop but also a description of a new species: P. gallaeciana. The strains displayed plant growth-promotion mechanisms, as well as production of hydrolytic enzymes, and could promote the growth of B. rapa, B. napus, B. nigra, B. juncea, and B. carinata. Remarkably, our results strongly suggest that progoitrin levels control fungal root colonization independently of the fungal nature, while the hydrolysis of sinigrin reduces colonization only in the case of endophytic fungi.
Data availability statement
The datasets presented in this study can be found in online repositories. The names of the repository/repositories and accession number(s) can be found in the article/Supplementary material.
Author contributions
PV and JP conceived the study. JP drafted the initial manuscript. PV, JP, and VR discussed the results and performed statistical analysis of the data. PV, JP, and FS carried out the fungi identification and phylogenetic analysis. MD-U maintained the fungal collection. ZS-S and EM performed the in vitro PGP assays. PV, VR, and MD-U performed the in planta PGP assays. JP and VR carried out the root colonization assays. PV and VR analyzed the GSLs profile. All authors contributed to the article and approved the submitted version.
Funding
This research was financially supported by projects RTI2018-096591-B-I00 34 (MCIU/AEI/FEDER, UE) and IN607A 2021/03 (Xunta de Galicia, Spain).
Acknowledgments
EM acknowledges an European Union’s Horizon 2020 Marie Sklodowska-Curie Actions (Grant Agreement nº 897795). Thanks to Dra. Alba Boscá from the University of Bristol-University of Salamanca for her advice on the choice of the new scientific name (Pyrenophora gallaeciana) based on Latin etymology.
Conflict of interest
The authors declare that the research was conducted in the absence of any commercial or financial relationships that could be construed as a potential conflict of interest.
Publisher’s note
All claims expressed in this article are solely those of the authors and do not necessarily represent those of their affiliated organizations, or those of the publisher, the editors and the reviewers. Any product that may be evaluated in this article, or claim that may be made by its manufacturer, is not guaranteed or endorsed by the publisher.
Supplementary material
The Supplementary material for this article can be found online at: https://www.frontiersin.org/articles/10.3389/fmicb.2022.981507/full#supplementary-material
Footnotes
References
Abdel-Farid, I. B., Jahangir, M., Mustafa, N. R., Van Dam, N. M., Van den Hondel, C. A., Kim, H. K., et al. (2010). Glucosinolate profiling of Brassica rapa cultivars after infection by Leptosphaeria maculans and fusarium oxysporum. Biochem. System. Ecol. 38, 612–620. doi: 10.1016/j.bse.2010.07.008
Al-Ani, L. K. T. (2019). “Recent patents on endophytic fungi and their international market” in Intellectual Property Issues in Microbiology. eds. H. B. Singh, C. Keswani, and S. P. Singh (Berlin, Germany: Springer). 271–284.
Amaike, S., Ozga, J. A., Basu, U., and Strelkov, S. E. (2008). Quantification of ToxB gene expression and formation of appressoria by isolates of Pyrenophora tritici-repentis differing in pathogenicity. Plant Pathol. 57, 623–633. doi: 10.1111/j.1365-3059.2007.01821.x
Anthony, M. A., Celenza, J. L., Armstrong, A., and Frey, S. D. (2020). Indolic glucosinolate pathway provides resistance to mycorrhizal fungal colonization in a non-host Brassicaceae. Ecosphere 11:e03100. doi: 10.1002/ecs2.3100
Bakri, Y., Arabi, M. I. E., and Jawhar, M. (2011). Heterogeneity in the ITS of the ribosomal DNA of Pyrenophora graminea isolates differing in xylanase and amylase production. Microbiology 80, 492–495. doi: 10.1134/S0026261711040023
Berbee, M. L., Pirseyedi, M., and Hubbard, S. (1999). Cochliobolus phylogenetics and the origin of known, highly virulent pathogens, inferred from ITS and glyceraldehyde-3-phosphate dehydrogenase gene sequences. Mycologia 91, 964–977. doi: 10.1080/00275514.1999.12061106
Card, S. D., Hume, D. E., Roodi, D., McGill, C. R., Millner, J. P., and Johnson, R. D. (2015). Beneficial endophytic microorganisms of brassica–a review. Biol. Control 90, 102–112. doi: 10.1016/j.biocontrol.2015.06.001
Chen, J., Ullah, C., Reichelt, M., Beran, F., Yang, Z. L., Gershenzon, J., et al. (2020). The phytopathogenic fungus Sclerotinia sclerotiorum detoxifies plant glucosinolate hydrolysis products via an isothiocyanate hydrolase. Nat. Commun. 11, 3090–3012. doi: 10.1038/s41467-020-16921-2
Ellwood, S. R., Liu, Z., Syme, R. A., Lai, Z., Hane, J. K., Keiper, F., et al. (2010). A first genome assembly of the barley fungal pathogen Pyrenophora teres f. teres. Genome Biol. 11, R109–R114. doi: 10.1186/gb-2010-11-11-r109
Eugui, D., Escobar, C., Velasco, P., and Poveda, J. (2022). Glucosinolates as an effective tool in plant-parasitic nematodes control: exploiting natural plant defenses. Appl. Soil Ecol. 176:104497. doi: 10.1016/j.apsoil.2022.104497
Farouk, H. M., Attia, E. Z., and El-Katatny, M. M. H. (2020). Hydrolytic enzyme production of endophytic fungi isolated from soybean (Glycine max). J. Mod. Res. 2, 1–7. doi: 10.21608/jmr.2019.15748.1008
Francisco, M., Tortosa, M., Martínez-Ballesta, M. D. C., Velasco, P., García-Viguera, C., and Moreno, D. A. (2017). Nutritional and phytochemical value of brassica crops from the Agri-food perspective. Ann. Appl. Biol. 170, 273–285. doi: 10.1111/aab.12318
Garcia-Fraile, P., Rivas, R., Willems, A., Peix, A., Martens, M., Martinez-Molina, E., et al. (2007). Rhizobium cellulosilyticum sp. nov., isolated from sawdust of Populus alba. Int. J. Syst. Evol. Microbiol. 57, 844–848. doi: 10.1099/ijs.0.64680-0
Hou, L., Yu, J., Zhao, L., and He, X. (2019). Dark septate endophytes improve the growth and tolerance of Medicago sativa and Ammopiptanthus mongolicus under cadmium stress. Front. Microbiol. 10:3061. doi: 10.3389/fmicb.2019.03061
Hu, X., Chen, J., and Guo, J. (2006). Two phosphate-and potassium-solubilizing bacteria isolated from Tianmu Mountain, Zhejiang, China. World J. Microbiol. Biotechnol. 22, 983–990. doi: 10.1007/s11274-006-9144-2
Hu, Z., Parekh, U., Maruta, N., Trusov, Y., and Botella, J. R. (2015). Down-regulation of fusarium oxysporum endogenous genes by host-delivered RNA interference enhances disease resistance. Front. Chem. 3:1. doi: 10.3389/fchem.2015.00001
Ismail, I. A., Godfrey, D., and Able, A. J. (2014). Proteomic analysis reveals the potential involvement of xylanase from Pyrenophora teres f. teres in net form net blotch disease of barley. Australas. Plant Pathol. 43, 715–726. doi: 10.1007/s13313-014-0314-7
Jiménez-Gómez, A., Saati-Santamaría, Z., Igual, J. M., Rivas, R., Mateos, P. F., and García-Fraile, P. (2019). Genome insights into the novel species microvirga brassicacearum, a rapeseed endophyte with biotechnological potential. Microorganisms 7:354. doi: 10.3390/microorganisms7090354
Jiménez-Gómez, A., Saati-Santamaría, Z., Kostovcik, M., Rivas, R., Velázquez, E., Mateos, P. F., et al. (2020). Selection of the root endophyte pseudomonas brassicacearum CDVBN10 as plant growth promoter for Brassica napus L. crops. Agronomy 10:1788. doi: 10.3390/agronomy10111788
Katoh, K., and Standley, D. M. (2013). MAFFT multiple sequence alignment software version 7: improvements in performance and usability. Mol. Biol. Evol. 30, 772–780. doi: 10.1093/molbev/mst010
Khalid, A., Arshad, M., and Zahir, Z. A. (2004). Screening plant growth-promoting rhizobacteria for improving growth and yield of wheat. J. Appl. Microbiol. 96, 473–480. doi: 10.1046/j.1365-2672.2003.02161.x
Khan, A. L., Hussain, J., Al-Harrasi, A., Al-Rawahi, A., and Lee, I. J. (2015). Endophytic fungi: resource for gibberellins and crop abiotic stress resistance. Crit. Rev. Biotechnol. 35, 62–74. doi: 10.3109/07388551.2013.800018
Kim, C. K., Seol, Y. J., Perumal, S., Lee, J., Waminal, N. E., Jayakodi, M., et al. (2018). Re-exploration of U’s triangle brassica species based on chloroplast genomes and 45S nrDNA sequences. Sci. Rep. 8, 1–11. doi: 10.1038/s41598-018-25585-4
Kliebenstein, D. J., Kroymann, J., Brown, P., Figuth, A., Pedersen, D., Gershenzon, J., et al. (2001). Genetic control of natural variation in Arabidopsis glucosinolate accumulation. Plant Physiol. 126, 811–825. doi: 10.1104/pp.126.2.811
Kubatko, L. S., and Degnan, J. H. (2007). Inconsistency of phylogenetic estimates from concatenated data under coalescence. Syst. Biol. 56, 17–24. doi: 10.1080/10635150601146041
Larsson, A. (2014). AliView: a fast and lightweight alignment viewer and editor for large data sets. Bioinformatics 30, 3276–3278. doi: 10.1093/bioinformatics/btu531
Letunic, I., and Bork, P. (2021). Interactive tree of life (iTOL) v5: an online tool for phylogenetic tree display and annotation. Nucleic Acids Res. 49, W293–W296. doi: 10.1093/nar/gkab301
Liu, Y., and Wei, X. (2019). Dark septate endophyte improves drought tolerance of Ormosia hosiei Hemsley & EH Wilson by modulating root morphology, ultrastructure, and the ratio of root hormones. Forests 10:830. doi: 10.3390/f10100830
Liu, Y. J., Whelen, S., and Hall, B. D. (1999). Phylogenetic relationships among ascomycetes: evidence from an RNA polymerse II subunit. Mol. Biol. Evol. 16, 1799–1808. doi: 10.1093/oxfordjournals.molbev.a026092
Madloo, P., Lema, M., Francisco, M., and Soengas, P. (2019). Role of major glucosinolates in the defense of kale against Sclerotinia sclerotiorum and Xanthomonas campestris pv. Campestris. Phytopathology 109, 1246–1256. doi: 10.1094/PHYTO-09-18-0340-R
Mateos, P. F., Jimenez-Zurdo, J. I., Chen, J., Squartini, A. S., Haack, S. K., Martinez-Molina, E., et al. (1992). Cell-associated pectinolytic and cellulolytic enzymes in rhizobium leguminosarum biovar trifolii. Appl. Environ. Microbiol. 58, 1816–1822. doi: 10.1128/aem.58.6.1816-1822.1992
Maximiano, M. R., de Jesus Miranda, V., de Barros, E. G., and Dias, S. C. (2020). Validation of an in vitro system to trigger changes in the gene expression of effectors of Sclerotinia sclerotiorum. J. Appl. Microbiol. 131, 885–897. doi: 10.1111/jam.14973
Mehmood, A., Irshad, M., Husna, A. A., and Hussain, A. (2018). In vitro maize growth promotion by endophytic fusarium oxysporum WLW. J. Appl. Environ. Biol. Sci. 8, 30–35.
Minh, B. Q., Schmidt, H. A., Chernomor, O., Schrempf, D., Woodhams, M. D., von Haeseler, A., et al. (2020). IQ-TREE 2: new models and efficient methods for phylogenetic inference in the genomic era. Mol. Biol. Evol. 37, 1530–1534. doi: 10.1093/molbev/msaa015
Murphy, B. R., Doohan, F. M., and Hodkinson, T. R. (2018). From concept to commerce: developing a successful fungal endophyte inoculant for agricultural crops. J. Fungi 4:24. doi: 10.3390/jof4010024
Nagaharu, U., and Nagaharu, N. (1935). Genome analysis in brassica with special reference to the experimental formation of B. napus and peculiar mode of fertilization. Jpn. J. Bot. 7, 389–452.
Newton, A. C., Fitt, B. D., Atkins, S. D., Walters, D. R., and Daniell, T. J. (2010). Pathogenesis, parasitism and mutualism in the trophic space of microbe–plant interactions. Trends Microbiol. 18, 365–373. doi: 10.1016/j.tim.2010.06.002
Onofre, S. B., Mattiello, S. P., da Silva, G. C., Groth, D., and Malagi, I. (2013). Production of cellulases by the endophytic fungus fusarium oxysporum. J. Microbiol. Res. 3, 131–134. doi: 10.5923/j.microbiology.20130304.01
Ortega, H. E., Torres-Mendoza, D., and Cubilla-Rios, L. (2020). Patents on endophytic fungi for agriculture and bio-and phytoremediation applications. Microorganisms 8:1237. doi: 10.3390/microorganisms8081237
Petit-Houdenot, Y., Degrave, A., Meyer, M., Blaise, F., Ollivier, B., Marais, C. L., et al. (2019). A two genes–for–one gene interaction between Leptosphaeria maculans and Brassica napus. New Phytol. 223, 397–411. doi: 10.1111/nph.15762
Petzel, J. P., and Hartman, P. A. (1986). A note on starch hydrolysis and β-glucuronidase activity among flavobacteria. J. Appl. Microbiol. 61, 421–426. doi: 10.1111/j.1365-2672.1986.tb04306.x
Plaszkó, T., Szűcs, Z., Cziáky, Z., Ács-Szabó, L., Csoma, H., Géczi, L., et al. (2022). Correlations between the metabolome and the endophytic fungal metagenome suggests importance of various metabolite classes in community assembly in horseradish (Armoracia rusticana, Brassicaceae) roots. Front. Plant Sci. 13:921008. doi: 10.3389/fpls.2022.921008
Posada, D. (2008). jModelTest: phylogenetic model averaging. Mol. Biol. Evol. 25, 1253–1256. doi: 10.1093/molbev/msn083
Poveda, J. (2021). Glucosinolates profile of Arabidopsis thaliana modified root colonization of Trichoderma species. Biol. Control 155:104522. doi: 10.1016/j.biocontrol.2020.104522
Poveda, J. (2022). Effect of volatile and non-volatile metabolites from Leptosphaeria maculans on tomato calli under abiotic stresses. Plant Stress 3:100054. doi: 10.1016/j.stress.2021.100054
Poveda, J., Abril-Urias, P., and Escobar, C. (2020c). Biological control of plant-parasitic nematodes by filamentous fungi inducers of resistance: Trichoderma, mycorrhizal and endophytic fungi. Front. Microbiol. 11:992. doi: 10.3389/fmicb.2020.00992
Poveda, J., and Baptista, P. (2021). Filamentous fungi as biocontrol agents in olive (Olea europaea L.) diseases: mycorrhizal and endophytic fungi. Crop Prot. 146:105672. doi: 10.1016/j.cropro.2021.105672
Poveda, J., Díaz-González, S., Díaz-Urbano, M., Velasco, P., and Sacristán, S. (2022). Fungal endophytes of Brassicaceae: molecular interactions and crop benefits. Front. Plant Sci. 13:932288. doi: 10.3389/fpls.2022.932288
Poveda, J., Eugui, D., Abril-Urías, P., and Velasco, P. (2021a). Endophytic fungi as direct plant growth promoters for sustainable agricultural production. Symbiosis 85, 1–19. doi: 10.1007/s13199-021-00789-x
Poveda, J., Eugui, D., and Velasco, P. (2020b). Natural control of plant pathogens through glucosinolates: an effective strategy against fungi and oomycetes. Phytochem. Rev. 19, 1045–1059. doi: 10.1007/s11101-020-09699-0
Poveda, J., Hermosa, R., Monte, E., and Nicolás, C. (2019). The Trichoderma harzianum Kelch protein ThKEL1 plays a key role in root colonization and the induction of systemic defense in Brassicaceae plants. Front. Plant Sci. 10:1478. doi: 10.3389/fpls.2019.01478
Poveda, J., Velasco, P., de Haro, A., Johansen, T. J., McAlvay, A. C., Möllers, C., et al. (2021b). Agronomic and metabolomic side-effects of a divergent selection for indol-3-ylmethylglucosinolate content in kale (Brassica oleracea var. acephala). Meta 11:384. doi: 10.3390/metabo11060384
Poveda, J., Zabalgogeazcoa, I., Soengas, P., Rodríguez, V. M., Cartea, M. E., Abilleira, R., et al. (2020a). Brassica oleracea var. acephala (kale) improvement by biological activity of root endophytic fungi. Sci. Rep. 10, 20224–20212. doi: 10.1038/s41598-020-77215-7
Rana, K. L., Kour, D., Sheikh, I., Yadav, N., Yadav, A. N., Kumar, V., et al. (2019). “Biodiversity of endophytic fungi from diverse niches and their biotechnological applications” in Advances in Endophytic Fungal Research. ed. B. P. Singh (Berlin: Springer), 105–144.
Rigobelo, E. C., and Baron, N. C. (2021). Endophytic fungi: a tool for plant growth promotion and sustainable agriculture. Mycology 13, 39–55. doi: 10.1080/21501203.2021.1945699
Rybak, K., See, P. T., Phan, H. T., Syme, R. A., Moffat, C. S., Oliver, R. P., et al. (2017). A functionally conserved Zn2Cys6 binuclear cluster transcription factor class regulates necrotrophic effector gene expression and host-specific virulence of two major Pleosporales fungal pathogens of wheat. Mol. Plant Pathol. 18, 420–434. doi: 10.1111/mpp.12511
Šamec, D., Urlić, B., and Salopek-Sondi, B. (2020). Kale (Brassica oleracea var. acephala) as a superfood: review of the scientific evidence behind the statement. Crit. Rev. Food Sci. Nutr. 59, 2411–2422. doi: 10.1080/10408398.2018.1454400
Sotelo, T., Velasco, P., Soengas, P., Rodríguez, V. M., and Cartea, M. E. (2016). Modification of leaf glucosinolate contents in Brassica oleracea by divergent selection and effect on expression of genes controlling glucosinolate pathway. Front. Plant Sci. 7:1012. doi: 10.3389/fpls.2016.01012
Stotz, H. U., Sawada, Y., Shimada, Y., Hirai, M. Y., Sasaki, E., Krischke, M., et al. (2011). Role of camalexin, indole glucosinolates, and side chain modification of glucosinolate-derived isothiocyanates in defense of Arabidopsis against Sclerotinia sclerotiorum. Plant J. 67, 81–93. doi: 10.1111/j.1365-313X.2011.04578.x
Szűcs, Z., Plaszkó, T., Cziáky, Z., Kiss-Szikszai, A., Emri, T., Bertóti, R., et al. (2018). Endophytic fungi from the roots of horseradish (Armoracia rusticana) and their interactions with the defensive metabolites of the glucosinolate-myrosinase-isothiocyanate system. BMC Plant Biol. 18, 1–15. doi: 10.1186/s12870-018-1295-4
Vandicke, J., De Visschere, K., Deconinck, S., Leenknecht, D., Vermeir, P., Audenaert, K., et al. (2020). Uncovering the biofumigant capacity of allyl isothiocyanate from several Brassicaceae crops against fusarium pathogens in maize. J. Sci. Food Agric. 100, 5476–5486. doi: 10.1002/jsfa.10599
Vela-Corcía, D., Srivastava, D. A., Dafa-Berger, A., Rotem, N., Barda, O., and Levy, M. (2019). MFS transporter from Botrytis cinerea provides tolerance to glucosinolate-breakdown products and is required for pathogenicity. Nat. Commun. 10, 2886–2811. doi: 10.1038/s41467-019-10860-3
Velasco, P., Rodríguez, V. M., Soengas, P., and Poveda, J. (2021). Trichoderma hamatum increases productivity, glucosinolate content and antioxidant potential of different leafy brassica vegetables. Plan. Theory 10:2449. doi: 10.3390/plants10112449
Vierheilig, H., Bennett, R., Kiddle, G., Kaldorf, M., and Ludwig-Müller, J. (2000). Differences in glucosinolate patterns and arbuscular mycorrhizal status of glucosinolate-containing plant species. New Phytol. 146, 343–352. doi: 10.1046/j.1469-8137.2000.00642.x
Vilgalys, R., and Hester, M. (1990). Rapid genetic identification and mapping of enzymatically amplified ribosomal DNA from several Cryptococcus species. J. Bacteriol. 172, 4238–4246. doi: 10.1128/jb.172.8.4238-4246.1990
White, T. J., Bruns, T., Lee, S. J. W. T., and Taylor, J. (1990). “Amplifcation and direct sequencing of fungal ribosomal rna genes for phylogenetics” in PCR Protocols: A Guide to Methods and Applications. eds. M. A. Innis, D. H. Garfield, J. J. Sninsky, and T. J. White (Cambridge: Academic Press), 315–322.
Witzel, K., Hanschen, F. S., Klopsch, R., Ruppel, S., Schreiner, M., and Grosch, R. (2015). Verticillium longisporum infection induces organ-specific glucosinolate degradation in Arabidopsis thaliana. Front. Plant Sci. 6:508. doi: 10.3389/fpls.2015.00508
Wu, X., Huang, H., Childs, H., Wu, Y., Yu, L., and Pehrsson, P. R. (2021). Glucosinolates in brassica vegetables: characterization and factors that influence distribution, content, and intake. Ann. Rev. Food Sci. Tech. 12, 485–511. doi: 10.1146/annurev-food-070620-025744
Keywords: sinigrin, Fusarium oxysporum, Setophoma terrestris, Acrocalymma vagum, Brassica U’s triangle, indoleacetic acid, glucosinolates
Citation: Poveda J, Rodríguez VM, Díaz-Urbano M, Sklenář F, Saati-Santamaría Z, Menéndez E and Velasco P (2022) Endophytic fungi from kale (Brassica oleracea var. acephala) modify roots-glucosinolate profile and promote plant growth in cultivated Brassica species. First description of Pyrenophora gallaeciana. Front. Microbiol. 13:981507. doi: 10.3389/fmicb.2022.981507
Edited by:
Mina Salehi, Tarbiat Modares University, IranReviewed by:
Rouhallah Sharifi, Razi University, IranSurinder Banga, Punjab Agricultural University, India
Siamak Farhadi, Tarbiat Modares University, Iran
Copyright © 2022 Poveda, Rodríguez, Díaz-Urbano, Sklenář, Saati-Santamaría, Menéndez and Velasco. This is an open-access article distributed under the terms of the Creative Commons Attribution License (CC BY). The use, distribution or reproduction in other forums is permitted, provided the original author(s) and the copyright owner(s) are credited and that the original publication in this journal is cited, in accordance with accepted academic practice. No use, distribution or reproduction is permitted which does not comply with these terms.
*Correspondence: Jorge Poveda, jorge.poveda@unavarra.es; Pablo Velasco, pvelasco@mbg.csic.es